Last Updated: October 23, 2024
Introduction to Hypoxia and Hypoxia Induced Factors
Hypoxia represents the pathological condition that exist when there is lower than normal oxygen content and pressure within cells. The response of cells to hypoxic conditions includes the activation of a family of transcription factors referred as hypoxia induced factors (HIF). There are three related HIF complexes identified as HIF-1, HIF-2, and HIF-3 that are defined by the particular α-subunit of the complex. The predominant HIF complex is HIF-1.
The HIF-1 pathway, which is activated by conditions of hypoxia (low oxygen tension), is a major homeostatic mechanism for cellular responses to changes in the level of oxygen within cells. HIF-1 is a heterodimeric complex composed of an α-subunit and a β-subunit. The β-subunit is constitutively expressed while the α-subunit expression and activity are increased in response to changes in cellular oxygen content. The activity of the HIF-1 and HIF-2 complexes are highly similar in their responses to hypoxia. Less detail is known regarding the HIF-3 complex which functions as a dominant-negative regulator of HIF activity.
Humans express three α-subunit genes, HIF1α (HIF1A gene), HIF2α (EPAS1 gene, for Endothelial PAS domain protein 1), and HIF3α (HIF3A gene). The PAS domain is so-called because of the three proteins in which the domain was originally identified: Per (period circadian protein), ARNT (aryl hydrocarbon receptor nuclear translocator), and Sim (simple-minded protein).
The HIF1A gene is located on chromosome 14q23.2 and is composed of 16 exons that generate three alternatively spliced mRNAs, each of which encode distinct protein isoforms. The HIF1A isoform 1 (826 amino acids) encoding mRNA represents the predominant HIF1A transcript. The HIF1A isoform 3 (850 amino acids) encoding mRNA is upregulated in activated T cells.
The EPAS1 gene is located on chromosome 2p21 and is composed of 17 exons that encode a protein of 870 amino acids.
The HIF3A gene is located on chromosome 19q13.32 and is composed of 18 exons that generate four alternatively spliced mRNAs, each of which encode distinct protein isoforms.
Expression of the HIF1A gene is ubiquitous, whereas expression of the EPAS1 (HIF2A) gene is more restricted being primarily found in interstitial cells, endothelial cells, and parenchymal cells. Expression of the HIF3A gene is found in numerous tissues with highest levels in adipose tissue, heart, spleen, and placenta.
The original β-subunit (HIF1β) was initially identified and characterized as ARNT. The ARNT gene is located on chromosome 1q21.3 and is composed of 25 exons that generate eight alternatively spliced mRNA, each of which encode distinct protein isoforms. Humans express two ARNT-related genes (ARNT2 and ARNTL), however, the encoded proteins are not components of the HIF complexes.
Transcriptional Regulation of HIF α-Subunit Genes
The oxygen-independent regulation of HIF activity is exerted via regulated transcription of the genes encoding the α-subunits. The generation of reactive oxygen species (ROS) is a major trigger for increased expression of HIF α-subunit genes. The effects of ROS are exerted via the activation of several kinases that includes PKC, PI3K, and IKK [inhibitor of nuclear factor kappa B (NFκB) kinase]. These kinases then phosphorylate and activate the transcription factors, NFκB which is a heterodimer encoded by the NFKB1 and NFKB2 genes, SP1 encoded by the SP1 gene, and NRF2 (nuclear factor erythroid 2–related factor 2) encoded by the NFE2L2 (NFE2 like bZIP transcription factor 2) gene. Growth factor signal transduction pathways also lead to increased transcription and translation of HIF-1α as a result of the activation of the PI3K and AKT kinases.
HIF α-Subunit Regulation via Prolyl Hydroxylation
The oxygen-dependent regulation of HIF activity is exerted via the α-subunits. Normally the HIF α-subunits are degraded in the presence of oxygen due to ubiquitylation. Ubiquitylation is a key modification directing proteins for rapid degradation by the proteasome machinery. The HIF α-subunits possess an oxygen-dependent degradation (ODD) domain. The ODD domain is hydroxylated by a member of the prolyl hydroxylase domain (PHD) family of proline hydroxylating enzymes.
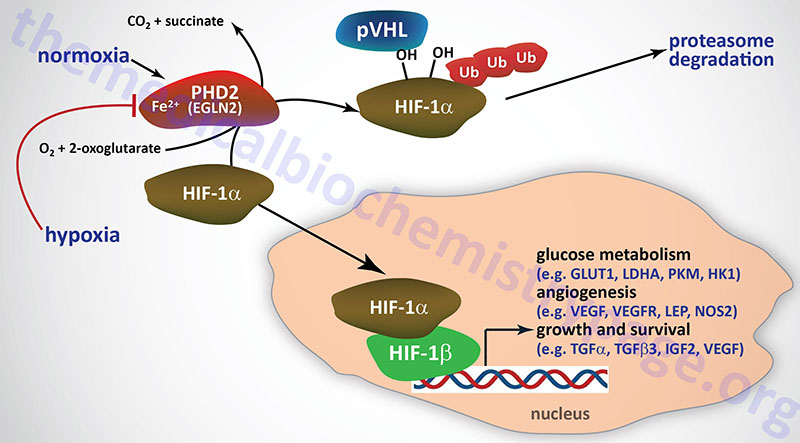
The prolyl hydroxylase family includes the enzymes that incorporate hydroxyl groups into proline residues in collagens of the extracellular matrix. The prolyl hydroxylases that hydroxylate HIF α-subunits are all ferrous (Fe2+) iron and 2-oxoglutarate (α-ketoglutarate)-dependent enzymes (2OG-oxygenases). The requirement of these enzymes for 2-oxoglutarate results in direct coupling of the activity this class of prolyl hydroxylases to metabolic processes that generate and utilize 2-oxoglutarate such as the TCA cycle.
The 2-oxoglutarate-dependent prolyl hydroxylase enzymes are identified as PHD1 (encoded by the EGLN2 gene), PHD2 (encoded by the EGLN1 gene) and PHD3 (encoded by the EGLN3 gene). The designation of EGLN refers to the fact that these three genes are homologs of the Caenorhabditis elegans egg laying-9 (Egl-9) gene. Hydroxylation of HIF α-subunits renders the proteins susceptible to proteasomal degradation under normoxic cellular conditions. In response to proline hydroxylation the ubiquitin ligase encoded by the von Hippel-Lindau (VHL) gene binds to the HIF α-subunit proteins and catalyzes their polyubiquitylation.
The PHD1 encoding gene (EGLN2) is located on chromosome 19q13.2 and is composed of 7 exons that generate two alternatively spliced mRNAs, both of which encode the same 407 amino acid protein.
The PHD2 encoding gene (EGLN1) is located on chromosome 1q42.2 and is composed of 5 exons that generate three alternatively spliced mRNAs, each of which encode a distinct protein isoform.
The PHD3 encoding gene (EGLN3) is located on chromosome 14q13.1 and is composed of 5 exons that generate two alternatively spliced mRNAs encoding proteins of 239 amino acids (isoform 1) and 145 amino acids (isoform 2).
Expression of PHD1 is highest in the testes with lower level expression seen in brain, liver, kidney, and heart. Expression of PHD2 is observed in most tissues. Expression of PHD3 is highest within cardiac myocytes and skeletal muscle. The activities of PHD2 and PHD3 are strongly induced by changes in oxygen concentrations. The hydroxylation reactions catalyzed by the PHD enzymes require molecular oxygen (O2) in addition to the Fe2+ and 2-oxoglutarate, therefore, reductions in oxygen content will result in loss of their activity. The products of the PHD enzymes are trans-4-hydroxyproline residues, CO2, and succinate.
The activity of the PHD enzymes is not only regulated by oxygen concentration but by numerous metabolites as well as by reactive oxygen species, ROS. ROS inhibit PDH activity allowing for stabilization of HIF1α and its migration to the nucleus. Similarly, metabolites that include pyruvate, lactate, succinate, and fumarate inhibit the activity of PDH enzymes. The significance of the metabolic inhibition of PDH activity relates to the altered metabolic profile of cancer cells and how this altered metabolism aids in the propagation of cancer cells, in part, through inhibition of HIF1α degradation. In addition to the normal metabolites that inhibit PDH enzymes, 2-hydroxyglutarate, that is formed as a result of the altered activity of isocitrate dehydrogenase 1 and 2 (IDH1 and IDH2) resulting from mutations in these genes, also inhibits the PDH enzymes. This latter process contributes to the cancers that develop in individuals harboring mutant IDH1 or IDH2 genes.
The activity of the HIF1α protein is regulated by being hydroxylated on two prolines (P405 and P531) in the ODD. The presence of the trans-4-hydroxyproline residues increases the binding of the VHL encoded protein (pVHL) by over 1000 fold. Given that the PHD enzymes require O2 as a substrate for the hydroxylation reaction, when conditions of hypoxia exist the HIFα subunits escape hydroxylation and are, therefore, not ubiquitylated. Under hypoxic conditions the stabilized HIFα subunits migrate to the nucleus and dimerize with the HIFβ subunit and activate the expression of target genes. Following interaction of pVHL, additional proteins are recruited that includes elongin C, elongin B, RBX1 (ring-box 1), cullin-2, and an E2 ubiquitin-conjugating enzyme forming a ubiquitin E3 ligase complex resulting in ubiquitylation of the HIF α-subunit and its subsequent degradation in the proteasome.
The activity of HIF1α is also regulated via the hydroxylation of a specific asparagine residue (N803) found in the C-terminal transactivation domain. The N803 hydroxylation is catalyzed by an enzyme of the 2-oxoglutarate and Fe2+-dependent dioxygenase family (2OG-oxygenase) originally identified as factor-inhibiting HIF-1 (FIH1; also identified as FIH). FIH1 is encoded by the HIF1AN (hypoxia inducible factor 1 alpha subunit inhibitor) gene. The consequences of the β-hydroxylation of N803 are that HIF1α can no longer interact with the transcriptional co-activators CBP [cAMP-response element-binding protein (CREB)- binding protein] and p300 (CBP/p300) resulting in inhibition of HIF-1 activity.
Additional Post-Translational Modifications of HIF α Subunits
Although hydroxylation by PHD family enzymes represents the predominant mechanism for post-translational regulation of HIF-1α activity and levels, the protein is also subject to acetylation/deacetylation, S-nitrosylation, and SUMOylation.
Numerous kinases phosphorylate HIF-1α, either directly or indirectly involving other targets of the kinases, resulting in modification of its activity in addition to those kinases discussed above that modify the expression of the HIF1A gene.
Several kinases including GSK-3β, p38MAPK, ERK1/2, CDK1 (cyclin-dependent kinase 1), and ATM (ataxia telangiectasia mutated) have been implicated in the direct phosphorylation HIF-1α. Most evidence clearly demonstrates a role for GSK-3β in the direct phosphorylation of HIF-1α resulting in stabilization of the protein and enhanced nuclear localization and transcriptional activation. Phosphorylation of HIF-1α by ERK1/2 also results in increased nuclear localization
Several kinases, including PI3K, AKT/PKB, PKA, AMPK, mTOR, and p70S6K have been shown to regulate the stability and activity of HIF-1αa by indirect mechanisms.
Transcriptional Regulation by HIF
As described in the Metabolic Alterations Associated with Cancer page, altered metabolism of glucose is a hallmark of all types of cancer. The diversion of glucose carbons into biomass in cancer cells necessitates an increased delivery of glucose into these cells and an increase in the rate of anaerobic glycolysis to lactate. This is accomplished by an increase in the expression of genes encoding glucose transporters and glycolytic enzymes. These transcriptional changes can be observed in over 70% of human cancers and is driven in part through activation of the hypoxia induced factor 1 (HIF-1) pathway and by increased expression of various proto-oncogenes and decreased expression of various tumor suppressors.
Both HIF1α and HIF1β proteins are transcription factors that contain basic helix-loop-helix domains that allow them to dimerize and to bind to DNA sequences in target genes. Both HIF1α and HIF1β also have a PAS domain. The HIF1α subunits also contain two transactivation domains (TAD), which regulate the expression of HIF-1 target genes. As indicated in the previous section, the transcriptional coactivator proteins CBP and p300 (CBP/p300) interact with HIF1α and this interaction occurs through the C-terminal TAD. CBP and p300 modify chromatin structure, and thereby transcriptional activity, via their lysine acetyltransferase (KAT) activity which acetylates the nucleosomal histones.
The normal role of the HIF-1 pathway is to promote the delivery of oxygen and nutrients to the oxygen-deprived tissue via the stimulation of neovascularization (angiogenesis). The microenvironment that surrounds most solid tumors is highly hypoxic and, therefore, the ability of the tumor cells to proliferate requires the ability to acquire oxygen and nutrients. This is accomplished, in large part, through the activation of the HIF-1 pathway which is considered to be a modulator in the transactivation of genes implicated in the altered metabolism observed in cancer cells.
HIF-1 Regulation of Glucose Homeostasis
Several of the metabolic regulatory genes, particularly of glucose metabolism, that are activated by HIF-1 include the pyruvate kinase M (PKM) gene, the phosphofructokinase 1 encoding (PFKL and PFKP) genes, the glucose-6-phosphate isomerase (GPI) gene, the glyceraldehyde-3-phosphate dehydrogenase (GAPDH) gene, the phosphoglycerate kinase 1 (PGK1) gene, the phosphoglycerate mutase (PGAM1) gene, the hexokinase 1 (HK1) and HK2 genes, the enolase 1 (ENO1) and ENO2 genes, the fructose-1,6-bisphosphate aldolase (ALDOA) gene, the pyruvate dehydrogenase kinase 1 (PDK1; also designated PDHK1) gene, the SLC2A1 gene (encoding GLUT1), the SLC2A3 gene (encoding GLUT3), the lactate dehydrogenase A (LDHA) gene, and the SLC16A3 gene (encoding the lactate transporter, monocarboxylate transporter 4: MCT4).
The altered patterns of metabolism lead to the accumulation of diverse metabolites in the microenvironment that promote tumor growth and contribute to the ability of the tumor cells to metastasize. Activation of HIF-1 in cancer cells results in restriction of glucose entry into the mitochondrial oxidative phosphorylation pathway via inhibition of the pyruvate dehydrogenase complex, PDHc. The inhibition of the PDHc is effected via HIF-1 stimulated expression of the PDK1 gene. The regulation of the PDHc by phosphorylation is discussed in detail in the Pyruvate Dehydrogenase and the TCA Cycle page.
The altered pyruvate kinase isoforms, as occurs in cancer, coupled with the activation of the HIF-1 pathway results in diversion of glucose metabolism into the pentose phosphate pathway as well as into increased lactate production. The conversion of pyruvate into lactate is enhanced in the context of activated HIF-1 since this transcription factor activates the expression of the LDHA gene. The increased production of lactate, by cancer cells, contributes to the acidification of the tumor microenvironment which, in turn, promote further activation of the HIF-1 pathway. Lactate accumulation also results in pyruvate accumulation in cancer cells. Pyruvate is a known inhibitor of the prolyl hydroxylases that hydroxylate the HIF1α subunit proteins. Loss of HIF1α proline hydroxylation results in increased HIF1α stability and, therefore, increased HIF-1 transcriptional activity. Thus, accumulation of lactate and pyruvate, which occurs as a result of both altered pyruvate kinase gene expression and activation of the HIF-1 pathway, further promotes activation of the HIF-1 pathway leading to a controlled and enhanced metabolic profile within cancer cells.
HIF-1 Regulation of Lipid Homeostasis
HIF-1 transcriptionally activates expression of the PPARG gene that encodes PPARγ. Increased expression of PPARγ results in enhanced synthesis of triglycerides (TG or TAG). HIF-1 also induces the expression the genes encoding fatty acid binding protein 3 (FABP3), FABP4, and FABP7 which contribute to the enhanced uptake of fatty acids under hypoxic conditions allowing for increased triglyceride synthesis. HIF-1 also enhances expression of the genes encoding the low-density lipoprotein receptor (LDLR) as well as the LDL receptor–related protein (LRP1). Increased expression of these lipoprotein receptors enables increased lipoprotein uptake under conditions of hypoxia.
HIF-1-mediated increases in fatty acid synthesis involve increased conversion of glutamine to 2-oxoglutarate (α-ketoglutarate) which, via the TCA cycle, provides the citrate necessary for transfer of mitochondrial acetyl-CoA from the mitochondria to the cytosol. HIF-1 activates expression of the gene encoding glutaminase 1 (GLS) which enhances glutamine metabolism.
HIF-1 activity enhances expression of the of the SREBF1 gene that encodes the SREBP-1 transcription factor. SREBP-1 controls the transcription of more than 30 genes whose encoded proteins are involved in the biosynthesis of cholesterol, triglycerides, phospholipids, and fatty acids. These genes include FASN (encoding fatty acid synthase) resulting in increased synthesis of fatty acids. Increased triglyceride synthesis results from HIF-1-mediated expression of AGPAT2 (encoding acylglycerol-3-phosphate acyltransferase 2) which converts lysophosphatidic acids (LPA) to phosphatidic acid (PA) through the addition of a fatty acid to the sn-2 (C2) position of an LPA. Phosphatidic acid is a substrate for triglyceride synthesis. HIF-1 also activates expression of the LPIN1 gene which encodes phosphatidic acid phosphatase identified as lipin-1. Lipin-1 removes the phosphate from PA to yield a diacylglyceride (DAG). Diacylglycerides also serve as substrates for triglyceride synthesis.
In contrast to enhanced fatty acid and triglyceride synthesis under hypoxic conditions, mediated by HIF-1, fatty acid oxidation is repressed under these conditions. Both HIF-1 and HIF-2 decrease expression of PGC-1α which is the transcriptional coactivator of several genes involved in β-oxidation. HIF-1 and HIF-2 also downregulate the expression of the CPT1A gene which encodes the out mitochondrial membrane carnitine palmitoyltransferase 1 (CPT1) which is required for transport of long-chain fatty acids into the mitochondria for oxidation.
HIF-1 Regulation of Reactive Oxygen Species Metabolism
The activation of HIF α-subunit gene expression by reactive oxygen species (ROS), as outlined above, is self limiting since HIF-1 activates expression of the gene (GCLM) encoding the regulatory subunit of the initial and rate-limiting enzyme of glutathione synthesis, γ-glutamylcysteine synthetase (also called glutamate-cysteine ligase, GCL). Glutathione is a major endogenous antioxidant crucial for the maintenance of the normal red-ox state of cells.
The GCLM gene encodes the protein identified as glutamate-cysteine ligase modifier subunit. The synthesis of glutathione begins with the amino acid glutamate whose intracellular levels are enhanced by the action of HIF-1. HIF-1 activity results in increased levels of glutamine transporters (e.g. SLC1A5 and SLC38A2) in the plasma membranes and also increases the level of glutaminase 1 (encoded by the GLS1 gene) that converts glutamine to glutamate.
HIF-1 also self limits the ROS mediated activation of its activity by regulating mitochondrial functions. When HIF-1 is active it modifies the activity of complex IV, specifically by activating the mitochondrial protease identified as Ion peptidase 1 (also known as LON). Ion peptidase 1 degrades one of the subunits of complex IV, the COX4I-1 subunit. While decreasing the amount of the COX4I-1 subunit, HIF-1 activity enhances the expression of the COX4I-2 subunit. This switch in COX4 subunit composition is associated with increased oxygen consumption which in turn reduces the likelihood for the production of ROS.