Last Updated: March 5, 2024
Introduction to Digestive Processes
The gastrointestinal (GI) system, with respect to the processes of food ingestion and digestion, comprises the hollow organs that include the oral cavity, the esophagus, the stomach, and the small and large intestines. At the gross level, the GI system can be divided into the upper GI and the lower GI. The upper GI includes the mouth, esophagus, and the stomach. The lower GI includes the small and large intestines and the rectum. In addition to these hollow organs, ingestion and digestion of food requires several accessory glands and other organs that add secretions to the hollow organs of the GI. The primary accessory organs necessary for digestion are the salivary glands present within the oral cavity, the pancreas, and the liver.
Table of the Major Locations of Digestive Processes
Organ | Major Digestive Function(s) |
oral cavity, salivary glands | generation of saliva to lubricate masticated food; secretion of salivary enzymes; carbohydrate digestion only |
stomach | production of HCl; secretion of digestive enzymes; protein and lipid digestion only; secretion of gastric hormone ghrelin |
pancreas | secretion of lipid and protein digestive enzymes; secretion of bicarbonate to neutralize gastric acid |
liver | production and secretion of bile acids required for lipid digestion in small intestine |
gallbladder | storage and concentration of bile acids |
small intestine | production of disaccharidases required for carbohydrate digestion; terminal digestion of carbohydrates, proteins, and lipids; absorption of nutrients and electrolytes |
large intestine (colon) | storage of digestive waste; absorption of water and electrolytes |
The GI organs are separated from each other at key locations by specialized structures termed sphincters. Entry of food from the oral cavity to the esophagus requires passage through the upper esophageal sphincter. The bolus of food then enters the stomach by passage through the lower esophageal sphincter. When food is propelled from the stomach into the duodenum of the small intestine it passes through the pyloric sphincter. Upon leaving the small intestines at the level of the ileum, digested food passes through the ileocecal valve into the large intestine, also referred to as the colon. Within the colon, waste is stored until it reaches the rectum which triggers nerve impulses to initiate defecation.
Each portion of the GI performs a specialized task in the overall process of digestion. The mouth begins the process by chopping and grinding the food into small pieces (mastication) while simultaneously lubricating it and initiating the process of carbohydrate digestion. When we swallow, the food is propelled by muscles in the esophagus past the lower esophageal sphincter into the stomach. The primary function of the esophagus is to serve as a conduit from the mouth to the stomach.
The processes of chewing and swallowing food are completely voluntary but all the rest of the events of digestion are carried out via involuntary processes. Once food enters the stomach it can be stored temporarily (in the upper stomach referred to as the fundus of the stomach) or further digested by stomach contractions that churn the food and mix it with gastric acid, gastric and lingual lipases, and the protease pepsin within the lower stomach.
Digestion continues in the small intestine after the digestive chyme is propelled from the stomach past the pyloric sphincter. The small intestine is also the major site for the absorption of nutrients in the food. The principal function of the large intestine is to reabsorb fluids and electrolytes and to store fecal matter prior to expulsion from the body in the process of defecation.
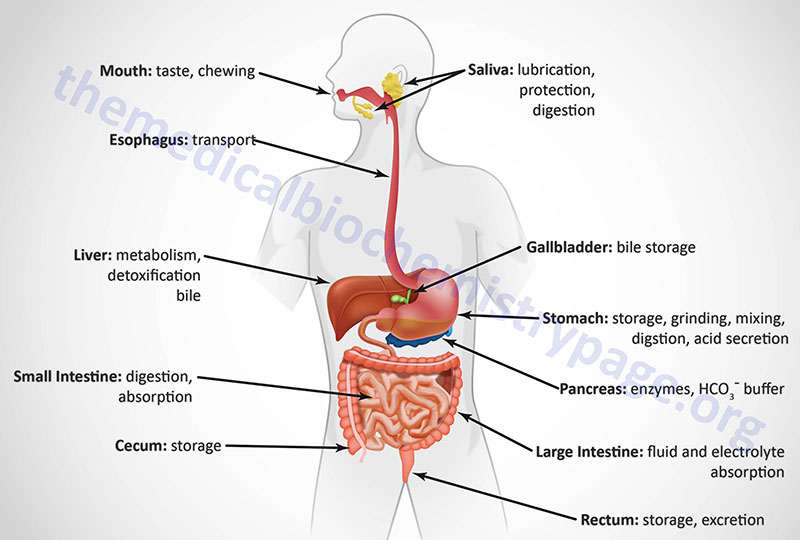
The Energy Derived from Digestion
When considering the amount of energy contained in various types of food components (e.g. fat, protein, carbohydrate) it is most important to understand that not all of the available energy can be delivered to the human body. This is due to several factors related to loss during digestive processes, incomplete absorption of nutrients, and there may also be non-digestible constituents in some foods. The absolute energy available in foods can be calculated by burning in a bomb calorimeter but this process reveals available energy in non-digestible fiber as well. Initial studies of conventional food energy were carried out in the late 19th century by the American chemist Wilbur Atwater but involved the complete burning of dried food in a bomb calorimeter.
The conventional assessment of energy value of a food takes into account the factors such as loss during digestion and absorption and thus, indicates the value of the food to the body as a fuel. The energy content of various food components is reported on product labels as either kilojoules/gram (kJ/g) or kilocalories/gram (kcal/g). Fat is the most energy-dense macronutrient, followed by alcohol, and then protein and carbohydrate. Amino acids from proteins, and the monosaccharides derived from dietary carbohydrates, contain more oxygen, relative to carbon and hydrogen, such that they are already partially oxidized and, therefore, yield less energy than fatty acids which are more reduced prior to their oxidation in the body.
Dietary fiber is not digested in the small intestines and therefore, imparts limited to no caloric content to the human diet. Dietary fiber is also called roughage and consists of both soluble and insoluble components. Soluble fibers are so-called because they dissolve in water. These are easily fermented by intestinal bacteria and are referred to as prebiotics. Insoluble fiber can also be fermented by intestinal bacteria and so can also be considered prebiotic. The advantage of insoluble fiber is that it absorbs water (particularly bulking fiber) as it transits the intestines softening the stool and thus, easing defecation. The fermentation of fiber by intestinal bacteria is known to be of physiological benefit since some of the products are absorbed and utilized for metabolic purposes such as the short-chain fatty acids (SCFA), acetate, propionate, and butyrate (see below).
Table of the Standard Energy Contribution from Various Components of Food
Component | Energy (kJ/gm) | Energy (kcal/gm) |
lipids (principally fatty acids) | 37 | 9 |
alcohol (specifically ethanol) | 29 | 7 |
protein | 17 | 4 |
carbohydrates | 17 | 4 |
organic acids | 13 | 3 |
sugar alcohols (sweeteners) | 10 | 2.4 |
fiber | 8 | 2 |
Digestive Processes of the Oral Cavity
Even before we place food in our mouth, our visual and olfactory senses trigger the release of salivary fluids in preparation for mixing with the food. The primary contents of saliva include water, enzymes, and electrolytes.
Table of the Major Components and Functions of Saliva
Saliva Component | Function(s): Comments |
water | major component comprising almost 99% of the volume of the saliva |
mucus | a mixture of mucopolysaccharides and glycoproteins high in sialic acid and sulfated sugars, mixes with water to form a lubricant for ease of swallowing |
electrolytes | sodium (Na+); potassium (K+); phosphate (PO43–); chloride (Cl–); bicarbonate (HCO3–); calcium (Ca2+); magnesium (Mg2+) |
α-amylase | secreted by acinar cells of the parotid and submandibular glands; initiates starch digestion into free glucose monomers |
lingual lipase | same enzyme as gastric lipase except within the oral cavity it is secreted by acinar cells of von Ebner glands; remains inactive until entering the acidic environment of the stomach where it functions along with gastric lipase (secreted by gastric Chief cells) to hydrolyze dietary triglycerides; functions only at acidic pH; active in the absence of bile acid emulsification as is required for pancreatic lipases |
lysozyme | antibacterial enzyme that hydrolyzes β-1,4-glycosidic linkages between N-acetylmuramic acid and N-acetyl-D-glucosamine in bacterial cell wall peptidoglycans |
kallikrein | secreted by acinar cells of all three major salivary glands, cleaves high molecular weight kininogen (HMWK) releasing the vasodilator, bradykinin |
transcobalamin 1 | commonly called haptocorrin; encoded by the TCN1 gene; binds vitamin B12 (cobalamin) to protect it from degradation in the acidic environment of the stomach, releases cobalamin in duodenum allowing it to be bound by intrinsic factor |
opiorphin | originally isolated from human saliva; encoded by the PROL1 (proline-rich, lacrimal 1) gene; the five amino acid functional peptide (QRFSR) is released from the N-terminus of the preproprotein encoded by PROL1; functions to reduce sensations of pain via its ability to inhibit the enkephalin-inactivating peptidases neprilysin [encoded by the membrane metallo-endopeptidase (MME) gene] and ecto-aminopeptidase N [encoded by the alanyl aminopeptidase, membrane (ANPEP) gene] |
antimicrobials | immunoglobulin; lactoperoxidase; lactoferrin; H2O2; thiocyanate |
Saliva is secreted from the parotid, submandibular, and sublingual glands within the oral cavity. There is a pair of parotid glands and these are the largest of the salivary glands. These glands are located posterior to the mandibular ramus and in front of the mastoid process of temporal bone. The parotid gland secretes saliva through Stensen’s ducts into the oral cavity. Parotid secretions are mainly serous in nature and facilitate mastication and swallowing. Enzymes (α-amylase) present in saliva are also responsible for the digestion of starches.
The submandibular glands are also a pair of glands. These glands are located beneath the lower jaw and superior to the digastric muscles which lie below the body of the mandible. Submandibular secretions are a mixture of both serous fluid and mucus that enters the oral cavity via Wharton’s ducts. Roughly 70% of the saliva present in the oral cavity is derived from the submandibular glands.
The sublingual glands are also a pair of glands that reside beneath the tongue and anterior to the submandibular glands. The salivary secretion produced by the sublingual glands is mainly mucus in nature. The sublingual glands do not have striated ducts like the parotid and submandibular ducts. Sublingual fluid is released from 8–20 excretory ducts and this fluid constitutes approximately 5% of saliva entering the oral cavity. The acidic lipase, originally referred to as lingual lipase, is secreted by lingual serous glands (von Ebner glands of tongue) and hydrolyzes medium- and long-chain triglycerides in the acidic environment of the stomach along with gastric lipase.
Chewing food is designed to chop, mash, and mix the contents of the mouth. The mixing of food and saliva represents the initial process of digestion. The action of α-amylase, on starch, releases glucose into the mouth. Glucose is sweet tasting which enhances the flavor of the food making it much more palatable and rewarding. When we swallow, the food is passed through the pharynx past the upper esophageal sphincter into the esophagus. Muscles in the esophagus then propel the food past the lower esophageal sphincter and into the stomach. The esophagus passes through the posterior mediastinum in the thorax and enters the abdomen through a hole in the diaphragm at the level of the tenth thoracic vertebrae. The esophagus is divided into three distinct sections referred to as cervical, thoracic and abdominal. As a consequence of the actions of the inferior pharyngeal constrictor muscle, entry to the esophagus occurs only when swallowing or vomiting.
Digestive Processes of the Stomach
The role of the stomach in overall digestive processes is complex and unique. The unique digestive functions of the stomach are related to its structure and to the types of secretory products produced by various specialized exocrine cells. The major secretory product of the stomach is gastric acid, HCl. In addition to acid, specialized cells of the stomach secrete the hormone ghrelin, water, mucus, bicarbonate, numerous anions and cations, intrinsic factor, and the zymogen pepsinogen. The stomach is tasked with storing ingested food, mixing ingested material with digestive juices and gastric acid, and then slowly emptying its contents past the pyloric sphincter into the duodenum of the small intestine.
In addition to being responsible for digesting food, the stomach also absorbs water-soluble and lipid-soluble substances. The digested contents of the stomach are referred to as chyme. Chyme is the semifluid material produced by the conversion of large solid particles into smaller particles via the combined peristaltic movements of the stomach and contraction of the pyloric sphincter. Chyme is generated by the propulsive, grinding, and retropulsive movements associated stomach peristalsis. The combination of the grinding action of the antrum, the retropulsion, and the squeezing of the chyme through the pyloric sphincter into the duodenum, is required for the emulsification of dietary fat and this plays an important role in the overall digestion and absorption of fat. In addition to digestion, the stomach also plays a critical role in the sensations of appetite and satiety. These latter functions are related to the hormone ghrelin as well as to vagal nerve responses to the stomach stretching (activation of mechanoreceptors) in response to uptake of the bolus of food from the esophagus.
Stomach Anatomy
Just as the GI system is divided into several specialized hollow organs, each of these hollow organs is anatomically and functionally subdivided. The stomach, from the lower esophageal sphincter to the pyloric sphincter, consists of three distinct domains based upon gross anatomy. As food passes through the lower esophageal sphincter it can be held in the upper stomach which is termed the fundus. The main chamber of the stomach, where digestion continues, is called the corpus of body of the stomach. The corpus is the largest anatomical subdomain of the stomach. The third subsection of the stomach resides at the lower portion of the organ just prior to the pyloric sphincter and is called the antrum.
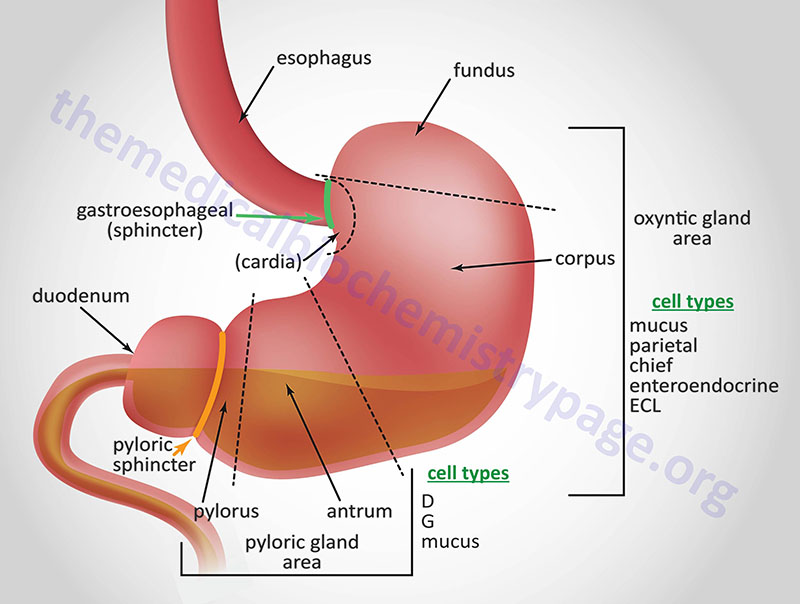
In addition to the three major anatomical domains of the stomach, the organ is also comprised of two functional areas, the oxyntic gland and the pyloric gland areas. The oxyntic gland area comprises 80% of the stomach and encompassing both the fundus and the corpus. The pyloric gland area encompasses the antrum. An area of the stomach just distal to the lower esophageal sphincter is sometimes referred to as the cardia but whether it represents a normal or pathophysiological domain is an area of debate.
The upper region of the stomach is called the fundus and this is where the bolus of food can be temporarily stored for up to an hour. The corpus is the largest segment of the stomach and the mucosa of this region contains many distinct exocrine and enteroendocrine cell types. The mucosal secretory cells of the fundus and corpus include mucus cells, parietal (oxyntic) cells, chief cells, and enteroendocrine cells. Chief cells are also called zymogen or peptic cells and are the cells that secrete the digestive protease precursor, pepsinogen. The primary function of mucus secretion by stomach epithelial cells is to protect the epithelium against shear stress and gastric acid. There are five or six distinct types of enteroendocrine cells in the mucosal layer of the stomach which includes the enterochromaffin-like cells (ECL) that secrete histamine, G cells that secrete gastrin, and A (X-like) cells that release the appetite stimulating hormone, ghrelin.
The last segment of the stomach is the antrum. The antrum is devoid of oxyntic glands and thus, the acid secreting parietal cells. The antrum (also called the pyloric antrum) contains both exocrine and enteroendocrine cells. The antrum enteroendocrine cells include G cells which secrete gastrin and D cells which secrete somatostatin. Both of these hormones are involved in regulated gastric acid production and secretion. Gastrin stimulates gastric acid production by parietal cells, whereas, somatostatin inhibits gastrin release from G cells and therefore, parietal cell acid secretions.
The wall of the stomach is itself composed of four distinct domains or layers. The gastric mucosa is the layer that lines the inside of the stomach. The subsequent layers include the submucosa, the muscularis externa, and then the serosa. The mucosal layer of the stomach is composed of an epithelium that harbors the various secretory glands. The submucosa consists of fibrous connective tissue that separates the mucosa from the muscularis externa. The muscularis externa of the stomach contains three layers of smooth muscle whereas, the rest of the GI tract only contains two layers of smooth muscle. The serosa is the outer layer of the stomach consisting of connective tissue that is continuous with the peritoneum.
The mucosal lining of the corpus of the stomach contains two major types of specialized glands, the pyloric and oxyntic glands. The oxyntic glands are composed of parietal cells that secrete gastric acid, exocrine cells (chief cells) that secrete pepsinogen, and mucus secreting cells. The acid secreting parietal cells are also called oxyntic cells. In addition, parietal cells of the fundus and corpus secrete intrinsic factor. Intrinsic factor is a glycoprotein that binds to vitamin B12 within the small intestine, facilitating its uptake by enterocytes of the distal ileum. Initially vitamin B12 is protected from gastric acid by binding salivary transcobalamin 1 (commonly called haptocorrin). After passing into the duodenum the transcobalamin 1 is degraded by pancreatic proteases and the vitamin is then bound to intrinsic factor.
The pyloric glands are located in the antrum segment of the stomach and contain cells that are responsible for secretion of gastrin (G cells), somatostatin (D cells), and mucus.
Gastric Acid Production
Gastric acid production and secretion is the major exocrine function of the stomach. The production and secretion of gastric acid (HCl), by oxyntic cells, as well as the zymogen pepsinogen, by chief cells, is controlled by both hormonal- and nerve-mediated processes. The nervous system components that impact the gastrointestinal tract (see below) are complex and consist of autonomic, parasympathetic (vagus), sympathetic (pre-vertebral ganglia), and enteric nerve fibers possessing both efferent (motor) and afferent (sensory) neurons. The enteric nervous system functions autonomously all along the GI tract as well as interacting with the CNS via vagal and pre-vertebral ganglia.
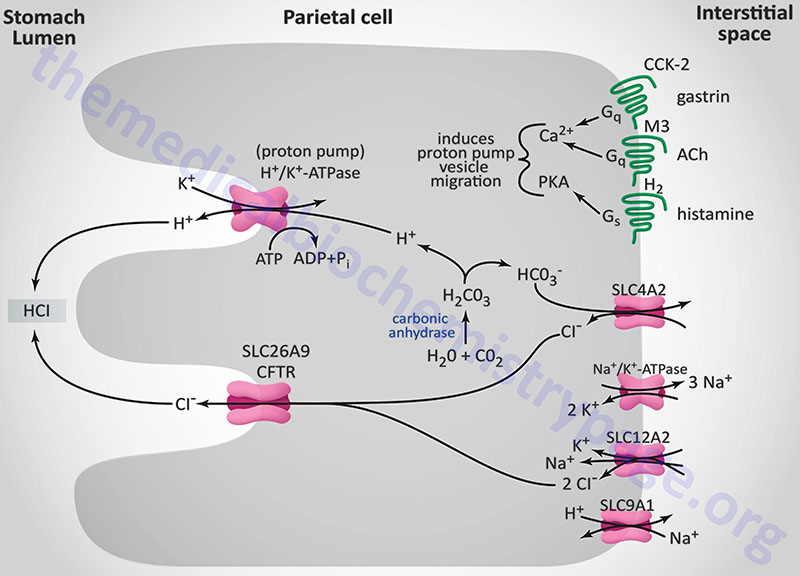
The principal stimulants for acid secretion are histamine (released from ECL cells), gastrin (released from G cells) and acetylcholine, ACh (released from postganglionic enteric neurons). Each of the three stimulators of acid secretion has different levels of functional importance, but histamine is thought to be the primary stimulus. The response of the stomach to the effects of ACh, gastrin, and histamine occur via a phenomenon referred to as potentiation. Potentiation is the effect observed where the response to two stimulants is greater than the sum of the effects of either stimulant alone.
Histamine is synthesized from the amino acid histidine via the action of histidine decarboxylase. Histamine is released from ECL cells in response to gastrin binding CCK-2 on these cells in stomach fundal glands. The released histamine then binds to histamine H2 receptors (H2R) on parietal cells. The H2R is a Gs-type GPCR that activates adenylate cyclase, leading to elevation of intracellular cAMP concentrations and activation of PKA. With respect to gastric acid production, PKA activation leads to phosphorylation of cytoskeletal proteins involved in transport of the H+/K+-ATPase (proton pump) from the cytoplasm localized membrane vesicles to the apical (luminal) plasma membrane of the parietal cell.
The gastric H+/K+-ATPase is a member of the P-type ATPase family of membrane transporters. The H+/K+-ATPase is the molecular pump of gastric acid secretion and it is solely responsible for the secretion of hydrogen ions into the lumen of the gastric glands and ultimately the stomach. The gastric proton pump is composed of a large α-subunit and a small highly glycosylated β-subunit. The composition of the H+/K+-ATPase is similar to the more ubiquitously distributed Na+/K+-ATPases. The α-subunit is encoded by the ATP4A gene, while the β-subunit is encoded by the ATP4B gene.
The ATP4A gene is located on chromosome 19q13.12 and is composed of 22 exons that encode a 1035 amino acid protein.
The ATP4B gene is located on chromosome 13q34 and is composed of 7 exons that encode a 291 amino acid protein.
The α-subunit carries out the ion exchange while the β-subunit stabilizes the α-subunit as well as preventing the pump from functioning in the reverse direction. The H+/K+-ATPase transports one hydrogen ion (H+) out of the cytoplasm of the parietal cell in exchange for one potassium ion (K+) obtained from the lumen of the gastric gland. The concentration of hydrogen ion in the lumen of gastric glands is such that the pH is on the order of 0.8, making it the most acidic environment in the human body.
The hydrogen ions (H+) are generated within the parietal cell from the dissociation of water. Water and CO2 are combined to form carbonic acid via the action of carbonic anhydrase. Carbonic acid rapidly ionizes to H+ and bicarbonate ion (HCO3–). This bicarbonate is then transported to the blood, across the parietal cell basolateral membrane, in exchange for chloride ion. The chloride ions are transported into the lumen of the gastric glands via the action of anion exchangers of the solute carrier (SLC) family or of the ATP-binding cassette (ABC) family. Gastric parietal cell chloride transport, into the lumen of the gastric glands, can occur via two different ion exchange complexes. One is encoded by the SLC26A9 gene the other by the cystic fibrosis transmembrane conductance regulator, CFTR gene (also designated the ABCC7 gene). Within the lumen of the gastric gland the hydrogen ions and chloride ions combine to form gastric acid (HCl). The CFTR gene is located on chromosome 7q31.2 and is composed of 27 exons that encode a 1480 amino acid glycoprotein.
Like all P-type ATPases, the gastric H+/K+-ATPase is able to transport ions against a concentration gradient by utilizing the energy derived from the hydrolysis of ATP. Indeed, the power of the gastric H+/K+-ATPase is such that it can transport H+ against a gradient in excess of 1,000,000 to 1. During ATP hydrolysis, a phosphate is transferred to the H+/K+-ATPase resulting in a conformational change in the pump. The conformational changes that occur to the H+/K+-ATPase in response to phosphorylation and dephosphorylation ensure that ion transport can only occur unidirectionally at any given point in time.
Gastric acid secretion occurs via three distinct phases in response to ingestion of food. These phases are termed the cephalic, the gastric, and the intestinal phases. Even though ingestion of food stimulates a large increase in gastric acid production, there is a small level, referred to as the basal level, of acid production at all times. The cephalic phase involves the CNS and is the result of responses to thinking of food, smelling food, and tasting food. These CNS responses transmit impulses to both parietal and enteroendocrine G cells (gastrin secreting) of the stomach via the vagal nerve. The vagal nerve endings contain acetylcholine (ACh) which, when released, binds to muscarinic-type (M3) receptors on parietal cells directly stimulating acid production and secretion. Acetylcholine can also indirectly stimulate gastric acid secretion by inhibiting the release of somatostatin through activation of M2 and M4 muscarinic receptors on D cells.
Vagal nerve endings on the stomach also contain gastrin-releasing peptide, GRP (a bombesin-like peptide also known as neuromedin B). The release of gastrin-releasing peptide triggers G cells to release gastrin. Gastrin then binds to receptors on parietal cells indirectly stimulating acid secretion. Gastrin exists in two major forms: little gastrin (17 amino acids) and big gastrin (34 amino acids) both of which result from a single precursor protein of 101 amino acid. Both forms of gastrin function by binding to the cholecystokinin receptor 2 receptor (CCK2: also called CCKB). The C-terminal tetrapeptide of both gastrins and cholecystokinin (CCK) are identical and possess all the biological activities of both gastrin and CCK. The cephalic phase accounts for 40% of total gastric acid secretion.
The distension (stretching) of the stomach as a result of food intake represents the gastric phase. The gastric phase of acid production is also stimulated by digestive products such as peptides. The distension of the stomach results in the stimulation of mechanoreceptors. Mechanoreceptor activation then causes parietal cell stimulation via both enteric (direct) and vagal nerve reflexes. Afferent and efferent impulses from the vagus nerve in the stomach mediate vago-vagal reflexes within the brain via the dorsal vagal complex. The gastric phase accounts for 50% of total gastric acid secretion.
The intestinal phase of gastric acid secretion only accounts for about 10% of the total gastric acid production. This phase is stimulated by protein digestion products within the duodenum. Circulating amino acids stimulate gastric acid secretion through their actions on the parietal cells.
Just as the production of gastric acid is physiologically significant with respect to digestion, the inhibition of gastric acid secretion is also physiologically significant. The secretion of gastric acid should only occur during digestion as it can damage the gastric and duodenal mucosa if inappropriately secreted or secreted in excess. Dysregulation of acid secretion is a significant cause of ulcerative conditions, therefore, the body has developed an elaborate system for regulating the amount of acid secreted by the stomach.
Gastric luminal pH is a sensitive regulator of acid secretion and protein in digested food is an excellent buffer. The buffering capacity of food maintains the luminal pH above 3.0. However, when the stomach is empty, or if acid production is excessive, gastric pH can fall below 3.0. When the pH falls (i.e. becomes more acidic), enteroendocrine D cells of the stomach antrum secrete somatostatin which inhibits gastrin release from oxyntic cells. In addition, somatostatin binds to the somatostatin 2 receptor (SSTR2) and SSTR4 receptors on parietal cells where the activation of the associated Gi-type G-protein leads to inhibition of the mobilization of gastric H+/K+-ATPase containing vesicles to the apical (luminal) membrane. This effect of somatostatin directly inhibits gastric acid production. Acidification of the duodenal lumen also triggers mechanisms to inhibit gastric acid production. Under low pH conditions duodenal enteroendocrine S cells release secretin which inhibits the release of gastrin.
In addition to digestion, the stomach also plays a critical role in the sensations of appetite and satiety. These latter functions are related to the hormone ghrelin as well as to vagal nerve responses to the stomach stretching in response to uptake of the bolus of food from the esophagus. As well as being responsible for digesting food, the stomach absorbs water-soluble and lipid-soluble substances. The digested contents of the stomach are referred to as chyme. Chyme is the semifluid material produced by the conversion of large solid particles into smaller particles via the combined peristaltic movements of the stomach and contraction of the pyloric sphincter. Chyme is generated by the propulsive, grinding, and retropulsive movements associated stomach peristalsis. The combination of the grinding action of the antrum, the retropulsion, and the squeezing of the chime through the pyloric sphincter into the duodenum is required for the emulsification of dietary fat and this plays an important role in the overall digestion and absorption of fat.
Clinical Consequences of Disturbed Gastric Acid Homeostasis
Disturbances in gastric acid homeostasis involve increases in both basal and stimulated gastric acid production. This disturbances to gastric homeostasis are the leading causes of ulcers in the mucosal lining of the gastrointestinal tract. These ulcers are collectively referred to as peptic ulcers. Four times as many peptic ulcers arise in the duodenum (called duodenal ulcers) versus the stomach itself (called gastric ulcers). The major cause of disrupted gastric acid homeostasis (accounting for as much as 70–90% of such ulcers) is infection with Helicobacter pylori (H. pylori), a helical-shaped bacterium that lives in the acidic environment of the stomach. Around 4% of all gastric ulcers are caused by malignant tumors called gastrinomas. Stress ulcers are most often found in the proximal stomach and result from mucosal ischemia and altered mucosal defense. Although duodenal ulcers are the result of increased acid secretion, gastric ulcers are more often associated with normal or decreased basal and stimulated acid production. Gastric ulcers are, therefore, most often caused by altered gastric mucosal defense which explains the propensity for NSAID-induced ulcers to occur in the stomach.
Gastric ulcers are classified into four groups according to their location and concomitant association with duodenal ulcer. Type I ulcers are found in the corpus and are generally characterized by low acid secretion, particularly at night. Type II ulcers occur in the antrum and are characterized by low, normal, or high acid secretion. Type III ulcers occur in close proximity to the pylorus and are associated with high acid output and duodenal ulcers. Type IV ulcers occur in the cardia and are characterized by low acid secretion. Treatment for gastric ulcers involves removing the injurious agent (e.g., NSAID or H. pylori) and inhibiting acid secretion. Antacids were the first therapeutic approach for ulcers. Unfortunately in order to neutralize luminal acid adequately, they need to be taken frequently which results in noncompliance and adverse effects. Although antacids with high neutralizing capacity, given after meals and at bedtime, can accelerate ulcer healing, pain is not significantly reduced.
The histamine H2 receptor antagonist (H2RA), cimetidine, was the first of a new class of peptic ulcer treatments. H2RA block both histamine-stimulated acid secretion and that induced by gastrin, which functions via stimulation of histamine release from ECL cells. The development of H+/K+-ATPase inhibitors (proton pump inhibitors, PPI), such as omeprazole, revolutionized the therapeutic management of gastric acid production. PPI directly inhibit the proton pump and therefore, they are capable of reducing both basal and stimulated gastric acid secretion independent of stimulus. PPI are much more effective than H2RA at reducing gastric acid production as well as being more effective in aiding the healing of duodenal ulcers and preventing their recurrence. In addition to these more common conditions that cause abnormal gastric acid production resulting in ulcers, there are several uncommon conditions.
The best characterized of these latter uncommon conditions is Zollinger-Ellison syndrome, ZES. ZES results from gastrin-producing tumors (gastrinomas) that result in hypersecretion of gastric acid. In any patient manifesting with refractory erosive esophagitis, multiple peptic ulcers, ulcers in the distal duodenum or jejunum, and recurrent ulcers after acid-reducing surgery, the possibility of ZES should be highly suspected. Patients with a family history of multiple endocrine neoplasia type 1 (MEN-1), or any of the endocrinopathies associated with MEN-1, that are presenting with multiple peptic ulcers should also be suspected of having ZES since approximately 25% of patients with ZES have MEN-1. MEN-1 is an autosomal dominant disorder characterized by pancreatic endocrine tumors, pituitary adenomas, and hyperparathyroidism. The PPI are used as the therapies of choice in the treatment of ZES, although very high doses are sometimes required to reduce the acid hypersecretion sufficiently.
Recently, gastroesophageal reflux disease (GERD) has become the most important acid-related clinical disorder. The pathogenesis of GERD involves acid in the wrong anatomical location, not excess acid production. Numerous treatments for GERD have been attempted but surgery is the most effective. Aside from surgery, the use of PPI are the most used drugs for treatment of GERD.
Digestive Processes of the Small Intestines
The intestines comprise a long hollow organ that runs from the stomach to the anus. The intestines are divided into three major anatomical and functional segments termed the small intestine, the large intestine, and the rectum. The small intestine is further divided into three functionally distinct segments. These segments, from the stomach to the large intestines are the duodenum, jejunum and ileum. The small intestines are around 20 feet in length with an average diameter of 1 inch. The large intestine, which is commonly referred to as the colon, is also subdivided into distinct segments. Beginning with the segment attached to the ileum these segments are the cecum, ascending colon, transverse colon, descending colon, and sigmoid colon. The large intestines are around 5 feet in length with an average diameter of 3 inches.
Similar to the layers of the stomach, the intestines are layered from inside the lumen to out with glandular epithelium and muscularis mucosa (together comprising the mucosal layer), sub mucosa, muscularis externa (only two layers whereas the stomach has three), and lastly the serosa. The muscularis externa, which is composed of both longitudinal and circular smooth muscles, provides the continuous peristalsis of the intestines that promotes the movement of digested food along the length of the gut to the rectum.
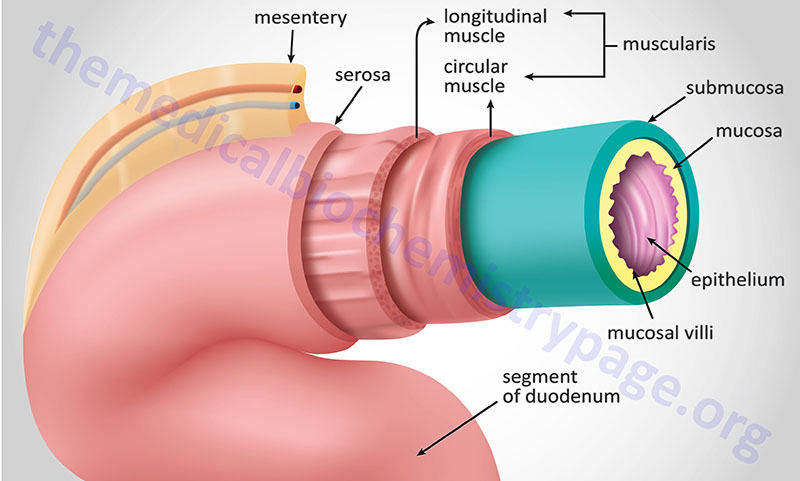
The primary function of the intestines is the digestion of food and the absorption of nutrients. Almost all of the nutrients in food, the amino acids, sugars, fats, and vitamins, are absorbed through the small intestines. The duodenum is the segment of the small intestine that receives the pancreatic digestive enzymes and pancreatic bicarbonate, and bile from the gallbladder via the ampulla of Vater. The epithelial cells of the duodenum also produces numerous enzymes required for efficient digestion such as enteropeptidase, and the various disaccharidases, aminopeptidase, dipeptidases, nucleotidases, and nucleosidases. The primary proteins/hormones released into the blood from the duodenum are cholecystokinin, secretin, and glucose-dependent insulinotropic peptide: GIP (GIP was originally identified as gastric inhibitory peptide). Absorption of nutrients is the primary function of the jejunum, while the ileum is responsible for absorption of vitamin B12 and bile salts. The ileum is the predominant site for the enteroendocrine L cells that secrete glucagon-like peptide 1 (GLP-1), GLP-2, protein tyrosine tyrosine (PYY), oxyntomodulin (OXM), and serotonin (5-HT).
The primary function of the large intestines is the resorption of water from food wastes thus creating fecal matter (the stool). The stool is then stored in the large intestines until it reaches the rectum where nerve impulses then stimulate the urge to defecate. In addition to water, the large intestines resorb sodium and any nutrients that may have escaped primary digestion in the ileum. The large intestine is also the site where gut bacteria (microbiota) are found. The importance of gut bacteria to human physiology is significant but in the context of digestion these microorganisms produce some vitamins and important nutrients and they digest molecules (particularly complex carbohydrates commonly referred to as non-digestible fiber) that cannot be degraded by human enzymes. Bacterial metabolism also accounts for the production of gases within the intestines.
Within the small intestine, the mucosal layer contains large numbers of invaginations termed villi. In addition, there are microvilli which dramatically increase the surface area of the small intestines and are the areas of the mucosal epithelium where nutrients are absorbed. In addition to the villi and microvilli, the small intestine contains numerous glands formed by pocket-like invaginations in the epithelium called crypts (crypts of Lieberkühn). Along the entire length of the intestinal glandular epithelium are goblet cells that produce mucus which lubricates the intestines as well as protects the tissue from digestive enzymes. The other major cell types in intestinal crypts and villi are the enterocytes and the enteroendocrine cells. Enterocytes are the cell types that absorb the nutrients in the gut. These cells also harbor the digestive enzymes that continue to digest food as it is being absorbed. Enterocytes contain a number of enzymes that are important for digestion but these enzymes remain associated with the brush-border membranes and are not released into the lumen of the gut. These enzymes include enteropeptidase, disaccharidases, aminopeptidases, dipeptidases, nucleotidases, and nucleosidases.
Nutrient Absorption in the Intestine
The absorption of nutrients from the gastrointestinal tract occurs predominantly within the small intestine while water and electrolyte transport occurs within the large intestine. All along the length of the small intestine, as well as within the large intestine, are numerous transport proteins that are responsible for the absorptive function of the gastrointestinal tract. These absorptive transporters reside within the apical (luminal) membrane of the intestinal enterocytes of the mucosal villi. The presence of villi dramatically increases the intestinal surface area by 30–600 fold. The intestinal villi are approximately 0.5–1.6 mm in length and are covered with columnar epithelial cells at the tip and crypt cells at the base. The columnar epithelial cells are primarily tasked with the absorptive functions of the intestine while the crypt cells are predominantly secretory.
The gastrointestinal tract processes approximately 8–10 L of fluid per day. On average this daily fluid volume contains approximately 800 mmol of sodium (Na+), 700 mmol of chloride (Cl–), and 100 mmol of potassium (K+). The bulk of this fluid and the electrolytes contained in it are absorbed through the small intestine, with approximately 1.5 L absorbed by the large intestine, and approximately 100 mL that is excreted in the stools. Transport of nutrients across the gastrointestinal epithelium is primarily the result of active transport processes.
Table of Nutrient Absorption from Various Intestinal Domains
Intestinal Segment | Nutrients Absorbed |
Duodenum | calcium; magnesium; sodium; chloride; ferrous iron (Fe2+), fat soluble vitamins; glucose, galactose, and fructose |
Jejuneum | heme iron; fats; fat soluble vitamins; sucrose; lactose; proteins; amino acids; glucose; water soluble vitamins |
Ileum | proteins; amino acids; cobalamin (vitamin B12), folate, and other water soluble vitamins; fat soluble vitamins |
Colon | H2O; potassium; sodium; chloride; proton (H+); bicarbonate; short chain fatty acids (SCFA) derived from colonic microbiota metabolism of non-digestible fiber |
Table of the Mechanisms of Intestinal Nutrient Absorption
Nutrient(s) | Absorptive Processes |
Vitamins | intestinal absorption of the individual water and fat soluble vitamins is covered in detail in each of the corresponding sections of the Vitamins: Water and Fat Soluble page |
Amino acids | intestinal absorption of the amino acids is covered in detail in the section below as well as in the Amino Acid Metabolism page |
Sugars | complex carbohydrates are degraded by various intestinal and pancreatic enzymes and the resultant monosaccharides are absorbed by the processes described in detail in the section below |
Fatty acids/lipids | intestinal absorption of the fatty acids and other dietary lipids is covered in detail in the section below as well as in the Lipolysis, Fat Mobilization, and Fatty Acid Oxidation page |
Short-Chain Fatty acids (SCFA) | the major intestinal short-chain fatty acids (acetate, propionate, and butyrate) are produced by bacterial fermentation of non-digestible fiber and other carbohydrates in the large intestine; SCFA absorption is primarily accomplished via the monocarboxylate transporter 1 (MCT1) encoded by the SLC5A8 gene; butyrate provides 60%–70% of the energy demands of enterocytes of the large intestine; luminal SCFA also activate numerous receptors on small intestinal enterocytes (FFAR2, FFAR3, HCA1, OR51E2) resulting in the activation of hormone secretion and numerous other processes in these cells as described in the section on Nutrient Sensing Receptors in the Small Intestine |
Sodium (Na+) | Na+-dependent amino acid and carbohydrate transporters; electroneutral Na+/H+ exchange (NHE transporters); epithelial Na+ channels (ENaC) in the large intestine; NHE transporters are members of the SLC9 family with NHE2 (SLC9A2 gene), NHE3 (SLC9A3 gene), and NHE8 (SLC9A8 gene) being responsible for intestinal Na+ absorption with NHE3 being the most significant; mutations in the SLC9A3 gene result in congenital sodium diarrhea; in the large intestine Cl–/HCO3– exchange (encoded by the SLC26A6 gene) along with Na+/H+ exchange contributes to Na+ absorption; ENaC transporters are composed of three subunits (α, β, and γ) where the α-subunit is encoded by the SCNN1a gene, the β-subunit by the SCNN1B gene, and the γ-subunit by the SCNN1G gene |
Chloride (Cl–) | chloride absorption occurs via passive (transcellular) and electroneutral transporter-mediated mechanisms; transport of Cl– involves coupling to Na+/H+ and Cl–/HCO3– exchange, as well as HCO3–-dependent Cl– absorption; the net effect of these transport processes is Na+ and Cl– absorption in exchange for H+ and HCO3– efflux to the lumen of the intestines; the same NHE transporters function in the processes of Cl– absorption |
Calcium (Ca2+) | Ca2+ uptake occurs via passive paracellular diffusion when at the normal high concentrations in the diet but by calcitriol-mediated processes at lower concentrations; paracellular diffusion primarily mediated by the intestinal epithelial tight junction associated claudin proteins encoded by the CLDN2, CLDN12, and CLDN15 genes whereas the CLDN5 encoded protein restrict diffusion of Ca2+; calcitriol-mediated Ca2+ absorption involves the induced expression of the calcium transporters encoded by the TRPV6 gene (encoding the transient receptor potential vanilloid 6 transporter), the SLC8A1 gene (encoding the Na+/Ca2+ exchanger 1; NCX1), and the ATP2B1 gene (encoding the plasma membrane Ca2+ transporter 1b; PCMA1b) |
Iron | ferrous (Fe2+) and heme iron both absorbed by intestine; dietary ferric (Fe3+) reduced to Fe2+ primarily via apical membrane associated duodenal brush border cytochrome b reductase 1 (DCYTB) which is encoded by the CYBRD1 gene; ferric reduction can also occur in the lumen via ascorbate; ferrous iron transported into enterocyte via divalent metal transporter 1 (DMT1) which is encoded by the SLC11A2 gene; heme iron is transported into jejunal enterocytes via heme carrier protein 1 (MCP1) which is encoded by the SLC46A1 gene; SLC46A1 also transports folate into enterocytes in a H+-coupled process |
Phosphate | inorganic phosphate (Pi) is primarily absorbed in the duodenum via Na+-dependent and calcitriol-dependent transport processes; passive paracellular transport contributes; calcitriol enhances apical Na+ phosphate cotransporter 2b (NPT2b, also designated NaPi-IIb) which is encoded by the SLC34A2 gene |
Magnesium (Mg2+) | primarily absorbed from the jejunum and ileum; passive paracellular transport via concentration gradients; heterodimeric transporter composed of transient receptor potential cation channel subfamily M, member 6 (TRPM6) and TRPM7 transport Mg2+ into enterocytes |
Sulfate (SO42–) | sulfate is absorbed predominantly via Na+-independent processes; sulfate anion transporter 1 (SAT1), encoded by the SLC26A1 gene, is major sulfate transporter in human intestine; the transporters encoded by the SLC26A2, SLC26A3, and SLC26A6 genes also contribute to intestinal sulfate absorption |
Zinc | absorption of zinc occurs throughout the small and large intestines with the majority of absorption occurring in the duodenum and jejunum; primary transporter is zinc transporter 4 (ZIP4) which is encoded by the SLC39A4 gene |
Copper | dietary cupric (Cu2+) copper is reduced to cuprous (Cu+) via the apical membrane associated DCYTB reductase as is the case for ferric iron reduction; the six-transmembrane epithelial antigen of the prostate 2 (STEAP2) protein has also been shown to reduce dietary cupric copper; Cu+ is transported into enterocytes by the apical high-affinity copper transporter 1 (CTR1) and CTR2 transporters which are encoded by the SLC31A1 and SLC31A2 genes, respectively |
Manganese (Mn2+) | the divalent metal transporter 1 (DMT1) transporter is primarily responsible for the absorption of manganese; metal iron transporters encoded by the SLC39A8 and SLC39A14 genes may also contribute |
Cadmium (Cd2+) | the divalent metal transporter 1 (DMT1) transporter is primarily responsible for the absorption of cadmium; metal iron transporters encoded by the SLC39A8 and SLC39A14 genes may also contribute |
Selenium (Se) | selenium exists in both inorganic and organic forms in nature; inorganic forms are selenate (SeO42–), selenite (SeO32–), selenide (Se2–), and elemental Se; major organic forms are (selenocysteine (SeCys) and SeMet; selenite, selenate, and SeMet are principal forms of selenium absorbed from the intestine; most likely absorbed via the action of the amino acid transporters encoded by the SLC1A4 and SLC3A1 genes |
Chromium (Cr3+) | intestinal absorption is via passive diffusion throughout the small intestine with predominantly uptake occurring in the jejunum |
Enteroendocrine Cells of the Digestive System
Within the epithelium of the gastrointestinal (GI) system resides a specialized subset of cells termed enteroendocrine cells (EEC). Enteroendocrine cells are dispersed throughout the GI epithelium yet they represent just 1% of the total cell population. Collectively these highly specialized cells for the largest endocrine system in humans. Enteroendocrine cells differ from other endocrine cells in that they function as single cells and have a very limited life span. Like other cells of the GI system epithelium enteroendocrine cells are being continuously renewed from stem cells that reside in the crypts of the mucosa.
A considerable amount of research has defined the organization of the enteroendocrine system so that it is now recognized that there are basically three distinct subsets of these cells: pan-GI tract, stomach selective and intestine selective enteroendocrine cell types. However, not all peptides are expressed in all cells at the same time throughout the GI system. Expression at the gene level and at the protein level can also be distinct. For example, within the proximal small intestine ghrelin expression is high but this is only detectable at the mRNA level. Although ghrelin peptide was originally thought to be exclusive to the stomach, it has been shown that a specialized enteroendocrine cell type of the small intestine co-expresses both motilin and ghrelin peptides.
Unlike the other gut-derived substance secreted from enteroendocrine cells, serotonin (5-hydroxytryptamin: 5-HT) is not a peptide but is derived from the amino acid tryptophan. The primary functions attributed to gut-derived serotonin were regulation of gastrointestinal motility and gastrointestinal secretory processes. However, recent evidence has shown that gut derived serotonin is capable of exerting multiple metabolic effects. These effects include adaptation to fasting by regulating lipolysis in adipose tissue and glucose uptake and gluconeogenesis in the liver. Gut derived serotonin also appears to be involved in processes of gastrointestinal inflammation, bone formation, and hepatic regeneration.
Table of Gastrointestinal Enteroendocrine Cells
Cell Type | Secreted Factor(s) | Major Location of Cells and Functions of Secreted Factors |
Stomach Enteroendocrine Cells | ||
A (X-like) cells | ghrelin, nesfatin-1 | predominantly located in the stomach ghrelin: induces increased feeding behavior nesfatin-1: derived via proteolytic processing of the 396 amino acid precursor protein encoded by the NUCB2 (nucleobindin 2) gene; reduces food intake |
Enterochromaffin-like (ECL) cells | histamine | specific to stomach; histamine binds to H2 type receptors to activate parietal cells of the stomach to secrete H+ for formation of HCl in lumen of stomach |
G cells | gastrin | predominantly located in the stomach antrum, some cells detected in duodenum; gastrin binds to cholecystokinin 2 (CCK2) receptors to activate parietal cells of the stomach to secrete H+ for formation of HCl in lumen of stomach; stimulates pepsinogen release from chief cells of stomach; also stimulates pancreatic secretions |
P cells | leptin | regulation of overall body weight by limiting food intake and increasing energy expenditure, regulation of the neuroendocrine axis, inflammatory responses, blood pressure, and bone mass; leptin is also a major white adipose tissue-derived adipokine |
Intestinal Enteroendocrine Cells | ||
D cells | somatostatin | considered pan-GI cell types; found in the stomach antrum and corpus as well as small intestine; somatostatin inhibits gastric acid production via antagonism of histamine release; inhibits pancreatic enzyme and bile acid secretions; inhibits release and action of numerous gut peptides such as CCK, OXM, PP, gastrin, secretin, motilin, GIP; |
Enterochromaffin (EC) cells | serotonin (5-HT) | considered pan-GI cell types; found in stomach, small intestine, and large intestine; acting primarily through 5-HT4 class receptors serotonin stimulates gastrointestinal motility and secretory processes |
I cells | cholecystokinin (CCK) serotonin (5-HT) | predominantly found in the proximal small intestine; CCK stimulates gallbladder contractions and bile flow; increases secretion of digestive enzymes from pancreas; vagal nerves in the gut express CCK1 (CCKA) receptors |
K cells | GIP serotonin (5-HT) | predominantly found in the proximal small intestine; glucose-dependent insulinotropic peptide (GIP) inhibits secretion of gastric acid; enhances insulin secretion; also referred to as gastric inhibitory peptide |
L cells | GLP-1 PYY oxyntomodulin (OXM) GLP-2 serotonin (5-HT) | primarily located in ileum (distal small intestine) and colon; small numbers have been found in duodenum and jejunum GLP-1: potentiates glucose-dependent insulin secretion; inhibits glucagon secretion; inhibits gastric emptying PYY: reduced gut motility; delays in gastric emptying, and an inhibition of gallbladder contraction; exerts effects on satiety via actions in the hypothalamus OXM: contains all of the amino acids of glucagon (see Figure below); inhibits meal-stimulated gastric acid secretion similar to GLP-1 and GLP-2 action; induces satiety, decreases weight gain, and increases energy consumption; has weak affinity for GLP-1 receptor as well as the glucagon receptor, may mimic glucagon actions in liver and pancreas GLP-2: enhances digestion and food absorption; inhibits gastric secretions; promotes intestinal mucosal growth |
M (Mo) cells | motilin | located in small intestine; distinct from the M cells of GI that are found in the lymph follicles termed Peyer’s patches; motilin initiates inter-digestive intestinal motility; stimulates release of pancreatic polypeptide (PP); stimulates gallbladder contractions |
N cells | neurotensin | located in jejunum; neurotensin a 13-amino acid peptide derived from a precursor that also produces neuromedin; involved in satiety responses and slows gastric emptying; also involved in nociception (sensation of pain) |
S cells | secretin | located in the duodenum and jejunum; secretin induces pancreatic bicarbonate secretion; inhibits gastric secretions; stimulates PP secretion |
Role of the Pancreas in Digestive Processes
The pancreas is a highly specialized organ found behind the stomach and it is surrounded by the small intestine, liver, and spleen. The organ is about six inches long and is largest at the end attached to the duodenum. This widest part is called the head of the pancreas, the middle section is called the neck and the body of the pancreas, and the thin end is called the tail.
The pancreas is composed primarily of two distinct types of secretory cells, endocrine and exocrine. The endocrine cells secrete a variety of hormones such as glucagon and insulin. The exocrine pancreas is involved in the secretion of numerous enzymes, electrolytes, and other substances involved in digestion. The exocrine pancreas has ducts that are arranged in clusters called acini (singular acinus). Pancreatic digestive secretions are expelled into the lumen of the acinus, and then accumulate in intralobular ducts that drain into the main pancreatic duct. The main pancreatic duct (pancreatic duct of Wirsung) joins the common bile duct coming from the gallbladder forming the ampulla of Vater. The ampulla is connected to the duodenal papilla through which the pancreatic juice, also containing bile, enters the small intestine.
Table of the Digestive Enzymes from the Pancreas
Enzyme | Activator | Function / Comments |
α-amylase (pancreatic) | chloride ion | activity is the same as salivary α-amylase |
carboxyl ester lipase, CEL | formerly call cholesterol esterase; hydrolyzes cholesteryl esters, tri-, di-, and monoglycerides, phospholipids, lysophospholipids, and ceramide | |
chymotrypsinogen | trypsin | cleaves proteins and peptides on the C-terminal side of aromatic amino acids, primarily Tyr, Pro, Trp |
colipase | trypsin | required at lipid-water interface for pancreatic lipase to function; the hormone enterostatin (which regulates fat intake) is derived from N-terminal end of pancreatic colipase; pentapeptide human enterostatin contains the sequence, APGPR |
deoxyribonuclease | hydrolyzes DNAs to free nucleotides | |
pancreatic lipase | hydrolyzes triglycerides to fatty acids and mono- and diacylglycerides; requires bile salt emulsified lipid droplets | |
procarboxypeptidase A (A1 and A2) | trypsin | cleaves proteins and peptides on the C-terminal side of aromatic and aliphatic amino acids |
procarboxypeptidase B | trypsin | cleaves proteins and peptides on the C-terminal side of aliphatic amino acids |
proelastase | trypsin | cleaves proteins and peptides on the C-terminal side of basic amino acids |
ribonuclease | hydrolyzes RNAs to free nucleotides | |
secreted phospholipase A2 (sPLA2) | trypsin | hydrolyzes the sn-2 position in phospholipids such as PE, PC, PI, PS releasing lysophospholipid (LPL) and a free fatty acid; at least 11 sPLA2 genes; pancreatic enzyme is encoded by the PLA2G1B gene; lysophosphatidylcholine (LPC, lyso-PC) is a bioactive proinflammatory lipid generated by the action of sPLA2 |
trypsinogen | enteropeptidase (also called enterokinase); embedded in duodenal mucosa | cleaves proteins and peptides on the C-terminal side of Arg and Lys residues |
In addition to enzymes described in Table above, the pancreas also secretes various cations, anions, water, and bicarbonate ion into the small intestine. Together with the pancreatic enzymes, the secretions constitute what is referred to as pancreatic juice. The cells of the pancreas tasked with electrolyte (e.g. bicarbonate) secretion are called duct cells. Pancreatic bicarbonate is critical to neutralizing the gastric acid entering the small intestine from the stomach. The production and secretion of pancreatic bicarbonate is essentially the same as for the process described for gastric acid secretion by parietal cells. Like parietal cell bicarbonate production, pancreatic bicarbonate is dependent on the enzyme carbonic anhydrase. The content of bicarbonate in pancreatic juice (around 115 mEq/L) is approximately 5-times higher than what is normally present in plasma. This high concentration of bicarbonate makes the pH of pancreatic juice around 8.0.
Exocrine glands of the pancreas also contain cells termed goblet cells. Goblet cells are tasked with the production and secretion of mucus into the pancreatic juice. Goblet cells are found nearest the distal end of the of pancreatic ducts and they can constitute as much as 25% of the epithelial cells at the distal end of the main pancreatic duct. Pancreatic mucus provides lubrication to the ducts as well as mechanical protection. In addition, the mucins (high molecular weight glycoproteins) present in pancreatic mucus serve an immunologic function by binding pathogens and interacting with immune cells.
Control of the secretory function of the acinar and duct cells of the pancreas is exerted via nervous and humoral mechanisms. The primary neural stimulus for pancreatic cell secretion of enzymes and bicarbonate is ACh released from postganglionic parasympathetic nerve endings. The primary hormonal triggers for acinar cell secretion are gastrin, cholecystokinin (CCK), and secretin. Gastrin is secreted by gastric G cells whereas, CCK is secreted by duodenal enteroendocrine I cells and secretin by S cells. All three hormones are secreted in response to the ingestion of food.
Cholecystokinin and ACh are the most important regulators of acinar protein secretion. Secretin is the most important regulator of pancreatic duct cell secretion of bicarbonate. The action of CCK on acinar cells exhibits a biphasic response in that induced secretion occurs at a particular dose of hormone but secretion is inhibited at higher doses. The CCKA receptor has the highest affinity for CCK and this receptor exists in both low affinity and high affinity states in acinar cells. Low concentrations of CCK activate the high affinity state receptor which induces acinar cell secretion, whereas, high concentrations of CCK activate the low affinity state receptor leading to inhibition of secretion. ACh activates acinar cell secretion by binding to muscarinic (M3) receptors.
As indicated earlier, the hormone secretin is involved in the response to acidification of the contents of the intestines. When the pH of intestinal fluid falls below around 4.5, S cells of the duodenum release secretin. At the level of the stomach secretin inhibits gastric acid production by inhibiting the release of gastrin and GIP. At the level of the pancreas, secretin stimulates pancreatic duct cells to secrete bicarbonate which when released into the duodenum increases the pH.
Role of the Gallbladder in Digestive Processes
The gallbladder is a small organ lying just beneath the liver between the costal margin and the lateral margin of the rectus abdominis muscle. The organ is divided into three sections called the fundus, body, and neck. The neck of the gallbladder tapers where it connects to the biliary tree via the cystic duct. The cystic duct then joins the common hepatic duct forming the common bile duct.
The gallbladder is the storage site for bile acids that were produced from cholesterol by the liver. The consumption of food, particularly lipid- and protein-rich foods, stimulates the release of CCK from duodenal enteroendocrine I cells which then enters the circulation and binds to receptors on the gallbladder. The binding of CCK to gallbladder cells induces pulsatile secretion of bile into the common bile duct which then meets with the pancreatic duct at the ampulla of Vater where they are secreted into the duodenum.
Bile salts are involved in the emulsification of lipids in the intestines aiding their digestion by the various pancreatic and duodenal lipases. Unused bile salts are returned to the gallbladder through the distal ileum and the portal circulation.
Carbohydrate Digestion and Absorption
Dietary carbohydrates enter the body in complex forms, such as monosaccharides, disaccharides, and polysaccharides with the primary polysaccharides being plant starch (amylose and amylopectin) and animal glycogen. The carbohydrate polymer cellulose is also consumed but not digested. Cellulose is referred to as indigestible fiber since the bacteria (microbiota) that inhabit the human intestinal tract do not possess the enzymes required to metabolize it.
The first step in the metabolism of digestible carbohydrate is the conversion of the higher polymers to simpler, soluble forms that can be transported into the intestinal enterocytes and then transported to the blood and delivered to the tissues. The breakdown of polymeric sugars begins in the mouth. Saliva has a slightly acidic pH of 6.8 and contains salivary α-amylase that begins the digestion of carbohydrates by hydrolyzing glucose present in α-1,4 glycosidic linkages. Saliva has a slightly acidic pH of 6.8 and contains salivary α-amylase that begins the digestion of carbohydrates. The action of salivary α-amylase is limited to the area of the mouth and the esophagus as it is virtually inactivated by the much stronger acid pH of the stomach.
The main polymeric-carbohydrate digesting enzyme of the small intestine is α-amylase. This enzyme is secreted by the pancreas and has the same activity as salivary α-amylase, producing disaccharides and trisaccharides. The latter are converted to monosaccharides by intestinal saccharidases, including maltase (maltase-glucoamylase) that primarily hydrolyzes di- and trisaccharides composed of glucose, and the more specific disaccharidases, sucrase-isomaltase, lactase (β-galactosidase), and trehalase. The net result is the almost complete conversion of digestible carbohydrate to its constituent monosaccharides.
Maltase-glucoamylase, as its name implies, has two distinct activities. The N-terminal domain of the enzyme possesses the maltase activity which exhibits highest activity towards the disaccharide of glucose termed maltose. The C-terminal domain has a broader specificity towards oligomers of glucose.
Maltase-glucoamylase is encoded by the MGAM gene. The MGAM gene is located on chromosome 7q34 and is composed of 75 exons that generate two alternatively spliced mRNAs encoding precursor proteins of 2753 amino acids (isoform 1) and 1857 amino acids (isoform 2).
Sucrase-isomaltase, as its name implies, has two distinct activities. The sucrase activity hydrolyzes sucrose into free glucose and fructose. Isomaltose is a disaccharide of two glucose molecules joined via an α-1,6 glycosidic bond. The primary source of isomaltase is through the action of a-amylase on starches since a-amylase cannot hydrolyze α-1,6 glycosidic bonds. The isomaltase activity hydrolyzes isomaltose into free glucose.
Intestinal sucrase-isomaltase is encoded by the SI gene. The SI gene is located on chromosome 3q26.1 and is composed of 51 exons that encode a precursor protein of 1827 amino acids.
Lactase is a member of the β-galactosidase family of enzymes and is active on numerous substrates. The primary substrate of lactase is lactose which it hydrolyzes to free galactose and glucose. The phlorizin hydrolase activity of the enzyme hydrolyzes glucose and galactose containing lactosylceramide, GM1 gangliosides, and β-galactoside containing glycoproteins.
Lactase is encoded by the LCT gene. The LCT gene is located on chromosome 2q21.3 and is composed of 17 exons that encode a 1927 amino acid preproprotein.
Trehalose is a disaccharide containing two glucose molecules linked via an α-1,1 glycosidic linkage. Trehalose is found in bacteria, fungi, plants, and invertebrates but not in mammals. Trehalase hydrolyzes glucose from trehalose.
Trehalase is encoded by the TREH gene. The TREH gene is located on chromosome 11q23.3 and is composed of 15 exons that generate two alternatively spliced mRNAs encoding precursor proteins of 583 amino acids (isoform 1) and 552 amino acids (isoform 2).
The net result of the action of the various digestive enzymes is the almost complete conversion of digestible carbohydrate to its constituent monosaccharides. The resultant glucose and other simple carbohydrates are transported across the intestinal wall to the hepatic portal vein and then to liver parenchymal cells and other tissues. Intestinal absorption of carbohydrates occurs via passive diffusion, facilitated diffusion, and active transport.
There are two intestinal transporters for glucose uptake from the lumen of the gut. One is sodium Na+-dependent [sodium-glucose transporter 1 (SGLT1)] while the other is Na+-independent [glucose transporter 2 (GLUT2)]. SGLT1 is the major transporter of glucose from the lumen of the small intestine. Although GLUT2 does indeed transport glucose into intestinal enterocytes, this only occurs in response glucose-mediated translocation of intracellular vesicle-associated GLUT2 thus, even in the absence of GLUT2 (such as is the case in individuals with Fanconi-Bickel disease), intestinal uptake of dietary glucose is unimpaired.
Galactose is also absorbed from the gut via the action of SGLT1. Fructose is absorbed from the lumen of the intestine via GLUT5 uptake. Indeed, GLUT5 has a high affinity for fructose and as such is considered a fructose-specific transporter.
Glucose uptake from the lumen of the gut and transepithelial transport to the portal circulation had been shown to occur via the action of two distinct glucose transporters. First, glucose is taken up from the intestinal lumen through the action of the sodium-dependent glucose transporter-1 (SGLT-1) then it is transported into the portal blood via the action of the facilitated glucose transporter GLUT2 present in the basolateral membrane. Evidence has also indicated that GLUT2 present in the apical (luminal) membrane of enterocytes was involved in glucose uptake. However, GLUT2 is not present in the apical membrane in the absence of a glucose load. The mechanism of GLUT2 presentation in the apical membrane involves a glucose-induced translocation of GLUT2 to this membrane. Thus, glucose uptake by the small intestine enhances additional uptake by promoting presentation of an additional transporter in the apical membrane.
Transport of galactose from the intestinal enterocyte to the circulation is the function of the basolateral membrane GLUT2 transporter. Transport of fructose from the intestinal enterocyte to the circulation is the function of the basolateral membrane-localized GLUT5 transporter.
Protein Digestion and Amino Acid Absorption
The digestion of dietary protein begins in the stomach and continues within the lumen of the duodenum. Within the small intestines there are two principal pancreatic enzymes involved in protein digestion; these are trypsin, and chymotrypsin. Several additional pancreatic peptidases play a lesser role in peptide digestion and include elastase and the carboxypeptidases.
The initial enzyme involved in protein digestion is gastric pepsin. The zymogen, pepsinogen, is secreted from chief cells in the stomach in response to the action of gastrin and to vagal nerve release of ACh. In fact there are at least eight isozymes of pepsinogen divided into two immunohistochemically distinct groups. Pepsinogens are activated to pepsins by stomach acid which alters their conformation freeing the normally inhibited catalytic domain. Once activated, pepsins will cleave 44 amino acids from pepsinogens generating more active pepsins. Pepsins hydrolyze peptide bonds on the C-terminal side of aromatic and hydrophobic amino acids. Approximately 20% of overall protein digestion is accomplished via the actions of pepsins. Due to the acidic pH optimum for the action of pepsins, these enzymes are inhibited when the chyme passes from the stomach and is mixed with alkaline pancreatic juices in the duodenum.
The remainder of protein digestion occurs within the duodenum and jejunum of the small intestine. Digestion here is primarily the result of the actions of trypsin and chymotrypsin, and to a lesser extent by elastase, and carboxypeptidases A and B, all of which are secreted by the pancreas. Trypsin is generated via the action of enteropeptidase action on pancreatic trypsinogen. Enteropeptidase is an enzyme secreted by cells of the crypts of Lieberkühn and resides in the brush-border membranes of duodenal mucosal cells. Trypsin then cleaves more trypsinogen to trypsin, as well as chymotrypsinogen, proelastase, and procarboxypeptidases to their active forms. In addition to enteropeptidase, intestinal brush border cells produce several other peptidases whose primary dietary functions are to digest tripeptides and dipeptides that are the result of pancreatic peptidase action. Of these intestine-derived peptidases aminopeptidase N (also known as alanine aminopeptidase) is of the most significance for digestion of small peptides to free amino acids.
Following digestion, free amino acids, as well as peptides (2-6 amino acids in length) are absorbed by enterocytes of the proximal jejunum. Some absorption also occurs in the duodenum and a minor amount in the ileum. Although there is little nutritional significance to whole protein absorption, some undigested dietary protein does get absorbed by intestinal enterocytes. Of significance is the fact that endogenous proteins, such as intestinal hormones and peptides, are absorbed intact. This uptake occurs primarily within the large intestines. The most significant function of intestinal whole protein absorption is in the neonate. During the first few days after birth neonates can absorb the immunoglobulins in colostrum (antibody-rich secretion from the mammary glands) allowing for the acquisition of passive immunity.
The absorption of amino acids requires an active transport process that is dependent upon either Na+ or H+ co-transport. There are several amino acid transporters encompassing seven distinct transport systems which are further grouped into three broad categories. There are the neutral amino acid (monoamino monocarboxylic) transporters, the dibasic (and cysteine) amino acid transporters, and the acidic (dicarboxylic) amino acid transporters. All of these transporters are members of the solute carrier (SLC) family of transporters. In all, a total of 36 amino acid transporters are expressed in humans, not all of which are found in the intestines. For a more complete overview of amino acid transporters go to the Amino Acid Biosynthesis and Catabolism page.
Intestinal uptake of peptides involves H+ co-transporters which are also members of the SLC family. The most abundant peptide transporter is PepT1 (encoded by the SLC15A1 gene) but there are three additional peptide transporters within the SLC15 subfamily. Within intestinal enterocytes the absorbed peptides are hydrolyzed to free amino acids via cytoplasmic peptidases. The free amino acids are then transported across the apical membranes of enterocytes and enter the portal circulation. Patients with Hartnup disorder, due to defective neutral amino acid transport, can remain asymptomatic on a high protein diet due to peptide absorption via PepT1.
Lipid Digestion and Absorption
The predominant form of dietary lipid in the human diet is triglyceride (TG or TAG). Gastrointestinal lipid digestion consists of several sequential steps that include physicochemical and enzymatic events. The physicochemical processes of lipid digestion involve the formation of large micelle aggregates or emulsion particles that consist of water and water-insoluble substrates. Within the stomach and the small intestines, the peristaltic actions of these organs aids in the emulsification of lipids. Bile acids secreted into the duodenum are also important for lipid emulsification.
The emulsified lipid droplets consist of a hydrophobic core containing the majority of the triglycerides, esterified cholesterol, and fat soluble vitamins. These droplets are surrounded by an amphipathic surface monolayer of phospholipids, free cholesterol, and a few triglycerides. Lipolysis occurs at the lipid-water interface of these particles. Efficient lipid digestion requires the formation of finely dispersed emulsions that have an interface composition favorable for the anchoring of lipases. The overall rate of intestinal lipolysis is, therefore, proportional to the available surface area of the fine emulsions. The enzymes involved in intestinal lipid digestion include gastric lipase and the pancreatic enzymes including pancreatic lipase, secreted phospholipase A (sPLA2), carboxyl ester lipase (CEL), as well as other acylglycerol hydrolases and phospholipases.
One of the first enzymes involved in the digestion of dietary lipids is lingual lipase which is secreted by serous (von Ebner) glands of the tongue. Lingual lipase remains essentially inactive, however, until it reaches the acidic environment of the stomach where this lipase hydrolyzes medium- and long-chain triglycerides. Within the stomach a second lipase, gastric lipase, is secreted by chief cells in the mucosa of the fundus of the stomach. Gastric lipase hydrolyzes primarily short- and medium-chain fatty acid linkages on the sn-3 position of triglycerides. The liberated medium-chain fatty acids can be absorbed directly in the stomach. Secretion of gastric lipase is stimulated by both hormonal and cholinergic mechanisms that involve gastrin, CCK, and ACh.
Lingual lipase and gastric lipase comprise the two acidic lipases. These lipases, unlike the alkaline lipases such as pancreatic lipase, are not dependent upon bile acids or colipase for maximal hydrolytic activity. Both of these acidic lipases account for up to 30% of total lipid hydrolysis that occurs during the digestive process. Gastric lipase is responsible for the bulk of the acidic lipase activity in the gut. In the neonate, acidic lipases account for as much as 50% of total lipid digestion.
Both lingual and gastric lipases are essential for the digestion of milk fat in the newborn because, unlike pancreatic lipase, they are able to penetrate fat globules in milk and initiate the digestive process. After passing from the stomach the digestion of fat continues in the duodenum with the synergetic action of gastric lipase and colipase-dependent pancreatic lipase. Colipase is secreted by the pancreas in an inactive form, procolipase and then activated in the intestinal lumen by trypsin.
Lipid digestion converts dietary fats into more polar derivatives with a higher degree of interaction with water facilitating their absorption by duodenal enterocytes. Triglycerides are converted to free fatty acids and glycerol via the action of gastric and pancreatic lipases. Cholesterol esters are hydrolyzed to free cholesterol and a free fatty acid via the action of carboxyl ester lipase (CEL). Phospholipids are hydrolyzed to lysophospholipids and a free fatty acid via the action of pancreatic sPLA2. The products of intestinal lipid digestion, mainly monoglycerides and ionized fatty acids, will leave the surface of an emulsified oil droplet and be incorporated into mixed micelles consisting of phospholipids and/or bile salts. A limited amount of lipid digestion also occurs when the micellar lipid particles come into contact with the membranes of the enterocytes.
Absorption of the digested lipid then occurs when these mixed micelles interact with enterocyte brush-border membranes. Fatty acid uptake by intestinal enterocytes occurs via protein-independent diffusion protein-dependent mechanisms. The fatty acid transport protein, FAT/CD36, plays a key role in the intestinal uptake of fatty acids. Another transport protein involved in facilitated fatty acid uptake is fatty acid transport protein 4, FATP4.
Carboxyl Ester Lipase and Cholesterol Uptake
Carboxyl ester lipase (CEL), like pancreatic lipase, is a major pancreatic enzyme involved in overall lipid digestion and absorption. CEL is also named pancreatic cholesterol esterase, cholesterol ester hydrolase, carboxylic ester hydrolase or bile salt-stimulated lipase. CEL functions over a wide pH range and is a non-specific lipid digesting enzyme capable of hydrolyzing cholesteryl esters, tri-, di- and monoglycerides, phospholipids, lysophospholipids, and ceramides. The hydrolytic activity of CEL towards water-insoluble triglycerides and cholesteryl esters requires its activation by bile salts, whereas, the activity of the enzyme in hydrolyzing water soluble substrates, such as lysophospholipids, is not dependent on bile salt activation. Only the cholesteryl ester hydrolytic activity of CEL is a substrate-specific function and is the only activity of this type in the digestive tract. Phospholipid hydrolysis in the gut can also be accomplished by pancreatic sPLA2, and initial triglyceride hydrolysis is accomplished principally via the activity of pancreatic lipase. However, pancreatic lipase hydrolyses triglycerides only to fatty acids and 2-monoacylglycerols. CEL is important for the complete digestion of acylglycerols to fatty acids and glycerol.
Only non-esterified cholesterol can be incorporated into micelles containing bile acids and it is these complexes that allow free cholesterol uptake by enterocytes. As indicated, cholesteryl esters are hydrolyzed by CEL allowing the free cholesterol to be incorporated into bile acid micelles. Upon interaction with the enterocyte membrane, several transport proteins have been shown to be important for cholesterol uptake. These transporters include the obligate heterodimer of ABCG5 and ABCG8.
The importance of ABCG5/ABCG8 in sterol uptake is demonstrated by the fact that mutations in either gene cause β-sitosterolemia in humans. This disorder is characterized by the accumulation of plant sterols in the blood and other tissues as a result of their enhanced absorption from the intestines and decreased removal in bile.
Another important cholesterol transporter is Niemann-Pick C1-like protein 1 (NPC1L1). The importance of NPC1L1 in cholesterol uptake was discovered with studies of the cholesterol absorption inhibitor ezetimibe which was shown to reduce diet-induced hypercholesterolemia.
The scavenger receptor, SR-BI, which is also the hepatic receptor for HDL is present in enterocyte basolateral membranes and has been shown to aid cholesterol uptake.
Nutrient Sensing Receptors in the Small Intestine
The nutrient-sensing receptors are members of the G-protein coupled receptor (GPCR) family that includes the taste (vomeronasal) receptors, the olfactory receptors, and a calcium sensing receptor family member. Although nutrient sensing through olfactory receptors and taste receptors occurs via structures in the head (nose and tongue), the gastrointestinal system is the major organ system for nutrient sensing. Indeed, members of the taste receptor family and the olfactory receptor family, as well as many other nutrient sensing receptors, are highly expressed in various cells of the gastrointestinal system. Not only do the gut nutrient sensing receptors sense and respond to nutrients in the diet they contribute to the secretion of the various gut-derived hormones.
Among the various nutrient-sensing GPCR of the intestine, the members of the large family of receptors that respond to free fatty acids are some of the most significant for modifying gut activities, gut-derived immune responses, and central nervous system responses.
Lipid Receptors for Long-Chain Fatty Acids (LCFA): The lipid-sensing receptors that bind LCFA include free fatty acid receptor 1 (FFAR1: GPR40) and free fatty acid receptor 4 (FFAR4: GPR120). FFAR1 is a receptor for long chain fatty acids (C12-C22) and several eicosanoids. FFAR1 is expressed on enteroendocrine I cells which secrete cholecystokinin (CCK) upon receptor activation. FFAR4 is expressed on enteroendocrine A (X-like) cells of the stomach that secrete ghrelin, on enteroendocrine L cells that secrete GLP-1, GLP-2, PYY, OXM, and serotonin, and on enteroendocrine I cells that secrete cholecystokinin (CCK). Expression is also high on pancreatic β-cells and is involved in the regulation of insulin secretion.
Lipid Receptors for Medium-Chain Fatty Acids (MCFA): The lipid-sensing receptors that bind MCFA include FFAR1, FFAR4, GPR84, and hydroxycarboxylic acid (HCA) receptor 3, HCAR3 (also known as GPR109B).
Lipid Receptors for Short-Chain Fatty Acids (SCFA): The lipid-sensing receptors that bind SCFA include FFAR2 (GPR43), FFAR3 (GPR41), HCAR1 (GPR109A), and olfactory receptor family 51 subfamily E member 2 (OR51E2; homolog of murine OLFR78 gene). The SCFA (acetate, propionate, and butyrate), the ligands of FFA2 and FFA3, are produced by the fermentation of ingested complex carbohydrates by intestinal bacteria. Sensing of the SCFA by these receptors, results in the modulation of a variety of physiological and hormonal processes that ultimately contribute to whole body energy sensing. FFAR2 is expressed on enteroendocrine A (X-like) cells of the stomach that secrete ghrelin and on enteroendocrine L cells that secrete GLP-1, GLP-2, PYY, OXM, and serotonin. FFAR3 is also expressed on enteroendocrine L cells. OR51E2 expressed in renal juxtaglomerular cells is responsible for renin release in response to SCFA. This effect of gut bacteria-derived SCFA on renal function plays an important role in overall regulation of blood pressure.
Lipid Receptors for Bile Acids: G-protein coupled bile acid receptor 1 (GPBAR1: also known as GPR131 or TGR5)
Lipid Receptor GPR119: GPR119 binds long-chain fatty acids including oleoylethanolamide (OEA), 2-oleoylglycerol (2OG), lysophosphatidylcholine (LPC or lysoPC), various lipid amides, and retinoic acid. The role of GPR119 in metabolic homeostasis is described in more detail in the Bioactive Lipids and Lipid Sensing Receptors page. The GPR119 gene is expressed on enteroendocrine L cells that secrete GLP-1, GLP-2, PYY, OXM, and serotonin. The GPR119 gene is a single exon intronless gene located on the X chromosome (Xq26.1) and encodes a protein of 335 amino acids.
Amino Acid Receptors: Receptors in the gut that bind amino acids include eukaryotic translation initiation factor 2 alpha kinase 4 (encoded by the EIF2AK4 gene; also known as GCN2), T1R1/T1R3 (taste type 1 receptors), GRM5 (glutamate metabotropic receptor 5), GPRC6A (member of the Ca2+ sensing receptor family along with CaSR), HCA3 (GPR109B). The GPCR of the T1R (sweet taste receptor type 1) and T2R (bitter taste receptor type 2) families involved in taste sensation are also expressed in the gut. The T1R family of receptors includes T1R1 (encoded by the TAS1R1 gene on chromosome 5p15.31), T1R2 (encoded by the TAS1R2 gene on chromosome 1p36.13), and T1R3 (encoded by the TAS1R3 gene on chromosome 1p36.33). The T2R family of receptors includes T2R1 (encoded by the TAS2R1 gene on chromosome 1p36.31) and T2R10 (encoded by the TAS2R10 gene located on chromosome 12p13.2). T2R receptors are responsible for sensation of bitter taste. T1R1/T1R3 heterodimer senses umami (umami taste stimulus is glutamate). T1R2/T1R3 heterodimer senses sweet. The G-proteins, gustducin (Ggust: encoded by the GNAT3 gene) and transducin (Gt: encoded by the GNAT1 gene), mediate downstream signaling of sweet, bitter, and umami tastes via interaction with T1R and T2R family receptors. Both gustducin and transducin activate PLCβ2 which leads to production of inositol-1,4,5-trisphosphate (IP3, Ins-1,4,5-P3) leading to release of endoplasmic reticulum (ER) stored Ca2+. The increased cytosolic calcium then activates TRPM5 (transient receptor potential subfamily M member 5; M refers to melastin)
Protein and Amino Acid (Peptone) Receptors: The lysophosphatidic acid receptor 5 (encoded by the LPAR5 gene; previously identified as GPR92/GPR93) activation results in activation of PLCβ2. LPAR5 is expressed in the majority of T1R1-positive taste cells indicating that umami cells may respond not only to amino acids but also to peptides. LPAR5 is expressed on enteroendocrine I cells that secrete cholecystokinin (CCK).
Monosaccharide Receptor: T1R2/T1R3 heterodimer recognizes all sweet taste stimuli. These taste receptors are expressed on enteroendocrine I cells that secrete cholecystokinin (CCK) and enteroendocrine L cells that secrete GLP-1, GLP-2, PYY, OXM, and serotonin.
Lactate Receptor: The gut receptor for lactate is hydroxycarboxylic acid (HCA) receptor 1 (HCAR1). Expression of HCAR1 is on enteroendocrine A (X-like) cells of the stomach that secrete ghrelin.
Calcium Sensing Receptors: The T1R1/T1R3 heterodimer recognizes the umami taste stimulus, L-glutamate. These taste receptors are expressed on enteroendocrine I cells that secrete cholecystokinin (CCK) and enteroendocrine L cells that secrete GLP-1, GLP-2, PYY, OXM, and serotonin.