Last Updated: September 10, 2024
Omega-3 and Omega-6 Polyunsaturated Fatty Acids (PUFA)
The term omega, as it relates to fatty acids, refers to the terminal carbon atom farthest from the functional carboxylic acid group (–COOH). The designation of a polyunsaturated fatty acid (PUFA) as an omega-3 fatty acid, for example, defines the position of the first site of unsaturation (carbon-carbon double bond) relative to the omega end of that fatty acid. Thus, an omega-3 fatty acid, such as α-linolenic acid (ALA), which harbors three carbon-carbon double bonds, has a site of unsaturation between the third and fourth carbons from the omega end. In addition to the position of the first site of unsaturation, relative to the omega end of an unsaturated fatty acid, the correct use of the omega nomenclature can only be applied to unsaturated fatty acids whose carbon-carbon double bonds are all in the cis orientation.
There are three major omega-3 fatty acids that are synthesized from precursor fatty acids, or ingested in foods, and then used by the body either directly or as precursors for bioactive lipids. These omega-3 fatty acids are ALA (an essential fatty acid; designated 18:3), eicosapentaenoic acid (EPA; designated 20:5), and docosahexaenoic acid (DHA; designated 22:6). There are numerous other omega-3 PUFA found in nature but for the purposes of this discussion, focus is placed on EPA and DHA.
Most of the ALA consumed in the diet comes from plant sources such as flax seed, walnuts, pecans, hazelnuts, and kiwifruit. There is a small percentage of omega-3 PUFA that come from meats common to Western diets such as chicken and beef, however, this is mostly ALA. The highest concentrations of EPA and DHA are found in cold water fishes such as salmon, tuna, herring, mackerel, and sardines. As discussed below (and in the Bioactive Lipid Mediators of Inflammation page), the most biologically significant omega-3 PUFA are EPA and DHA.
Another omega-3 fatty acid, whose biological activities are under investigation, is docosapentaenoic acid (DPA; designated 22:5). DPA can exist in both an omega-3 fatty acid form and an omega-6 fatty acid form. The omega-3 form (designated DPAn-3) is all-cis-7,10,13,16,19-docosapentaenoic acid (trivial name clupanodonic acid). As shown in the Figure below DPAn-3 is formed from EPA. The omega-6 form (designated DPAn-6) is all-cis-4,7,10,13,16-docosapentaenoic acid (trivial name: osbond acid). DPAn-6 is formed from arachidonic acid.
Eicosatetraenoic acid (ETA designated 20:4) represents another important PUFA that, like DPA, can exist in an omega-3 or an omega-6 form. The omega-3 form (designated ETAn-3) is all-cis-8,11,14,17-eicosatetraenoic acid. This PUFA is designated 20:4 in the Figure below and is formed from stearidonic acid (18:4). The omega-6 form (all-cis-5,8,11,14-eicosatetraenoic acid), which is ETAn-6, is more commonly called arachidonic acid.
EPA and DHA are the two primary omega-3 PUFA that serve as bioactive lipids themselves by binding to and activating the G-protein coupled receptor (GPCR) first identified as GPR120. The GPR120 protein is encoded by the free fatty acid receptor 4 (FFAR4) gene. In addition to activating a transmembrane GPCR, EPA and DHA are known to bind and activate members of the nuclear receptor family, specifically peroxisome proliferator activated receptor-alpha (PPARα) which is activated by DHA and PPARγ which is activated by EPA.
EPA and DHA also serve as important precursors for lipid-derived modulators (members of the oxylipin family) that are known to contribute to the prevention of cardiovascular diseases, to exert anti-inflammatory effects, to reduce the level of circulating lipids (hypolipidemic effect) while simultaneously increasing lipid catabolism via the activation of the transcriptional co-activator, PPARα, and to reduce the likelihood for non-alcoholic fatty liver disease, NAFLD. NAFLD is now referred to as metabolic dysfunction-associated fatty liver disease (MAFLD).
There are many vegetarian and vegan sources of omega-3 PUFA but these sources contain ALA. Although ALA can serve as the precursor for EPA and DHA synthesis in humans, this pathway (see Synthesis of Omega-3 and -6 Fatty Acids section) is highly limited in its capacity and also varies between individuals. Therefore, direct dietary intake of omega-3 fats rich in EPA and DHA are of the most benefit clinically. Outside the context of fortified foods, algae are the best natural sources of EPA and DHA for vegetarians and vegans.
Additional Important Omega Unsaturated Fatty Acids
In the consideration of biologically significant fatty acids, the omega-3 and omega-6 fatty acids, although highly clinically significant, should not completely overshadow the facts that there are two additional omega fats of clinical significance. These additional fatty acids are the monounsaturated fatty acids (MUFA), palmitoleic acid (16:1) and oleic acid (18:1). Palmitoleic acid is an omega-7 MUFA and oleic acid is an omega-9 MUFA.
Palmitoleic acid is likely to be a potent anti-aging fat. Experimental and clinical studies have shown that palmitoleic acid exerts many of its beneficial effects via activation of the master regulatory enzyme called AMP-activated protein kinase, AMPK.
Oleic acid serves as the precursor for the potent bioactive lipid ethanolamide, oleoylethanolamide (OEA). OEA exerts potent effects within the central nervous system to control feeding behavior and in the pancreas to increase glucose-stimulated insulin secretion (GSIS).
Punicic acid is an 18 carbon PUFA that is classified as a conjugated linolenic acid. The oil extracted from pomegranate seeds is very nearly 100% punicic acid. Punicic acid contains three carbon-carbon double bonds, two that exist in the cis orientation and one in the trans orientation. Because punicic acid is a PUFA it is often erroneously classified using the omega fatty acid nomenclature and as such is often identified as an omega-5 PUFA. However, as indicated above, the correct use of the omega PUFA nomenclature can only be applied to unsaturated fatty acids whose carbon-carbon double bonds are all in the cis orientation.
Despite the confusion regarding its naming, punicic acid has been shown to have potent anti-oxidant activities, to prevent obesity in laboratory animals, and to reduce the negative effects of the metabolic syndrome in humans. Of significance to the health benefits of punicic acid, pomegranate juice has little of this important PUFA and the seeds are discarded after juice extraction for retail sale of pomegranate juice.
Synthesis of Omega-3 and -6 Fatty Acids
Most of the omega-6 PUFA consumed in the diet is from vegetable oils such as soybean oil, corn oil, safflower oil, and borage oil, and consists of the 18-carbon (18:2) PUFA linoleic acid. Linoleic acid, which is an essential fatty acid, is converted to arachidonic acid through the steps outlined in the Eicosanoid Metabolism: Prostaglandins, Thromboxanes, Leukotrienes, and Lipoxins page.
The synthesis of omega-3 fatty acids, EPA and DHA, utilizes the other essential fatty acid, α-linolenic acid (ALA), which is also an 18-carbon (18:3) PUFA. The overall pathway for EPA and DHA synthesis involves three endoplasmic reticulum (ER) fatty acid elongation enzyme systems, three desaturation reactions involving two different desaturases, and a peroxisomal β-oxidation step as the final step in DHA synthesis as outlined in the Figure below.
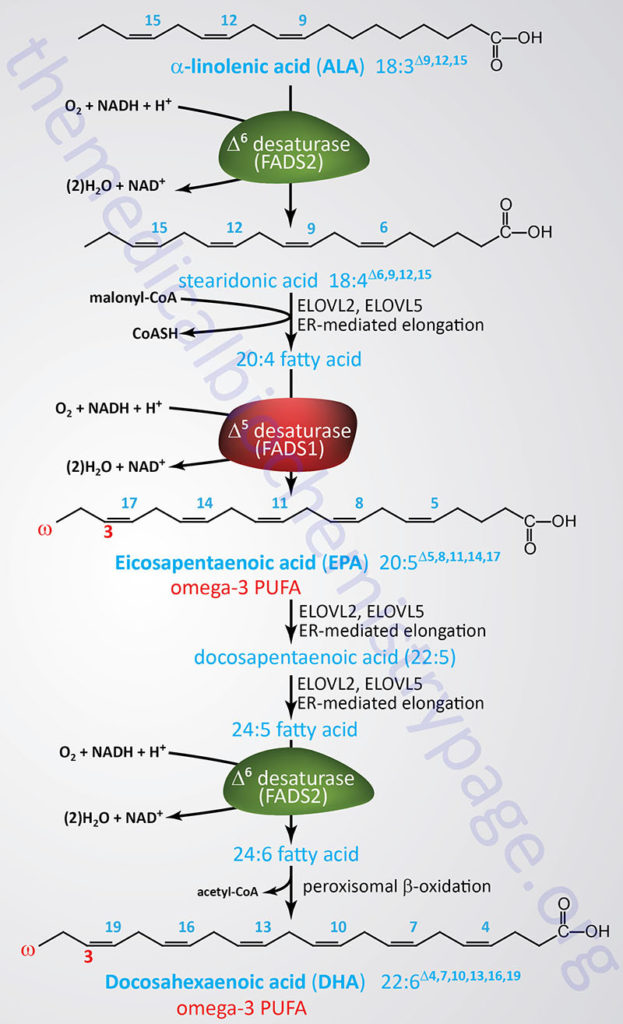
The desaturases involved in EPA and DHA synthesis are Δ5-eicosatrienoyl-CoA desaturase (D5D) and Δ6-oleoyl(linolenoyl)-CoA desaturase (D6D). As the name of these enzymes implies the D5D enzyme catalyzes the desaturation of the C5 to C6 bond in fatty acids while the D6D enzyme catalyzes the desaturation of the C6 to C7 bond.
The D5D enzyme is encoded by the FADS1 gene. The FADS1 gene is located on chromosome 11q12.2 and is composed of 13 exons that encode a protein of 501 amino acids.
The D6D enzyme is encoded by the FADS2 gene. The FADS2 gene is located on the same chromosome as the FADS1 gene, 11q12.2 and is composed of 13 exons that generates three alternatively spliced mRNAs that encode three distinct isoforms of the enzyme.
The 11q12.2 chromosomal region is referred to as the FADS cluster and harbors an additional desaturase gene identified as FADS3. The FADS3 encoded enzyme functions as a ceramide desaturase in the synthesis of ceramides, particularly in the synthesis of 4,14-sphingadiene (SPD).
The activity of both the D5D (FADS1) and D6D (FADS2) enzymes is slow and can be further compromised due to nutritional deficiencies as well as during inflammatory conditions. In healthy females only around 20% of dietary ALA is converted to EPA and less than 10% is converted to DHA. In healthy males only around 8% of ALA is converted to EPA and there is no appreciable conversion to DHA. The primary limitation in the de novo DHA synthesis pathway is the conversion of DPA to DHA.
The rate of EPA and DHA conversion from ALA is reduced by up to 40% when ALA is consumed along with pro-inflammatory omega-6 fatty acids, such as the essential fatty acid linoleic acid and arachidonic acid, as is typical in the Western-style diet. In addition, the consumption of alcohol has been shown to reduce the level of DHA in the liver and the blood. In addition, hyperglycemia and hypercholesterolemia are both known to interfere with the activity of D5D and D6D. For these reasons it is highly recommended that dietary supplementation with natural food sources of EPA and DHA, as well as purified versions of these omega-3 fatty acids, is instituted.
Mechanisms of Omega-3 Fatty Acid Biological Activities
The metabolic and clinical significances of the omega-3 PUFA are due to the ability of these lipids to directly exert effects on numerous biologically important pathways. In addition to the direct action of the omega-3 fatty acids EPA and DHA, and also to some extent DPA, there are numerous metabolites of these fatty acids that are classified as bioactive lipids. The most potent bioactive lipids that are derived through various enzymatic processes acting on EPA and DHA are the lipoxins, resolvins, protectins, and maresins. The synthesis of the lipoxins and their biological activities are covered in more detail in the Bioactive Lipid Mediators of Inflammation page. The precise mechanisms by which these PUFA, and their metabolites, exert their effects have only recently begun to be elucidated.
Several G-protein coupled receptors (GPCR), originally identified as GPR40, GPR41, GPR43, GPR84, and GPR120 have been shown to be activated by free fatty acids (FFAs). Short-chain fatty acids are specific agonists for GPR41 and GPR43 and medium-chain fatty acids for GPR84. Long-chain fatty acids can activate GPR40. Because these GPCR have been shown to be activated by free fatty acid binding their names have been changed to free fatty acid receptor 1 (FFAR1: GPR40), FFAR2 (GPR43), FFAR3 (GPR41), and FFAR4 (GPR120). FFAR1, FFAR2, and FFAR3, their ligands, and their biological activities are described in greater detail in the Bioactive Lipids and Lipid Sensing Receptors page.
FFAR4/GPR120 Activities
Since FFAR4/GPR120 is directly activated by DHA and EPA its role in the functions of these omega-3 fatty acids is discussed here. Stimulation of FFAR4/GPR120 by omega-3 PUFA has been shown to result in elevation of intracellular Ca2+ levels and activation of the extracellular signal-regulated kinase (ERK) family cascade as a result of the associated Gq– and G11-type G-proteins.
FFAR4/GPR120 is highly expressed in adipose tissue, and proinflammatory macrophages. The high expression level of FFAR4/GPR120 in mature adipocytes and macrophages is indicative of the fact that FFAR4/GPR120 is likely to play an important role in biologic functions of these cell types. In contrast, negligible expression of FFAR4/GPR120 is seen in muscle, pancreatic β-cells, and hepatocytes. Although not expressed at appreciable levels in hepatocytes expression of FFAR4/GPR120 is highly inducible in liver resident macrophage-like cells known as Kupffer cells. FFAR4/GPR120 can be activated with a synthetic agonist (GW9508) as well as the omega-3 PUFA, EPA and DHA. FFAR4/GPR120 is also expressed in enteroendocrine L cells of the gut. These are the cell types that express the incretin peptide hormone GLP-1. Previous work on FFAR4/GPR120 focused on the potential ability of this receptor to stimulate L cell GLP-1 secretion.
Short-chain fatty acids are known to be proinflammatory and unsaturated fatty acids are generally neutral. In contrast the omega-3 PUFA, DHA and EPA, exert potent anti-inflammatory effects through FFAR4/GPR120. It has been found that GPR120 functions as an omega-3 fatty acid receptor/sensor in proinflammatory macrophages and mature adipocytes. By signaling through FFAR4/GPR120, DHA and EPA, mediate potent anti-inflammatory effects to inhibit both the Toll-like receptor (TLR) and tumor necrosis factor-α (TNFα) inflammatory signaling pathways. The TLRs are a class of non-catalytically active transmembrane receptors that are involved in mediating responses of the innate immune system. Their name is derived from the fact that they have sequence similarities to the Toll gene found in Drosophila.
It is known that chronic tissue inflammation is an important mechanism resulting in the development of insulin resistance. Therefore, the anti-inflammatory actions of omega-3 PUFA can exert potent insulin sensitizing effects. Experiments utilizing an obese mouse model demonstrated that the in vivo anti-inflammatory and insulin sensitizing effects of omega-3 PUFA are dependent on expression of FFAR4/GPR120. Given that FFAR4/GPR120 is highly expressed in proinflammatory macrophages and functions as an omega-3 PUFA receptor, this receptor is likely to be a critical component of the anti-inflammatory effects of the omega-3 PUFA class of lipids.
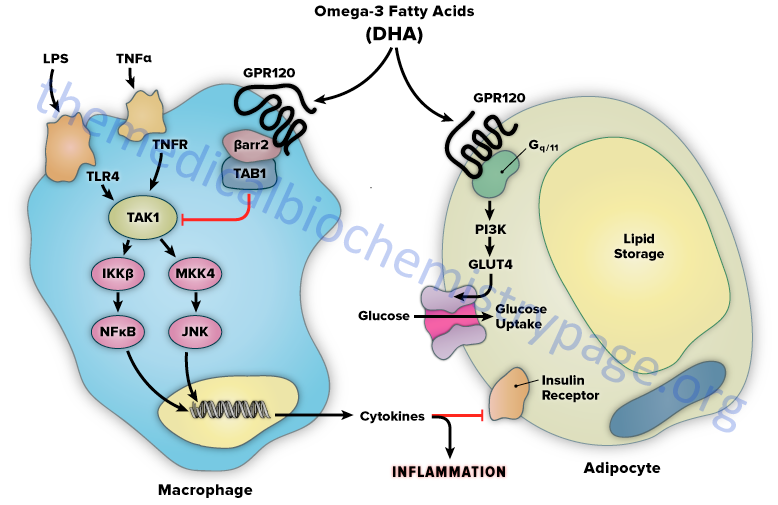
The mechanism of FFAR4/GPR120-mediated anti-inflammation involves inhibition of transforming growth factor β–activated kinase 1 (TAK1) through a β-arrestin-2 dependent effect. β-arrestins are a class of protein that serve as scaffold or adaptor proteins for a wide range of GPCR, as well as several other groups of receptor subtypes. After ligand binding, β-arrestins can associate with the cytoplasmic domains of GPCRs and couple the receptor to specific downstream signaling pathways, as well as mediate receptor endocytosis.
Stimulation of FFAR4/GPR120 by DHA has been shown to inhibit both the TLR2/3/4 and TNFα proinflammatory cascades. Activation of the kinases, inhibitor of nuclear factor-kappa B kinase subunit beta (IKKβ) and c-JUN N-terminal kinase (JNK), is common to TLR and TNFα signaling. Nuclear factor-kappa B (NF-κB) is one of the most important transcription factors regulating the expression of proinflammatory genes. Given that activation of FFAR4/GPR120 by DHA results in inhibition of both the TLR and TNFα cascades it indicates that the locus of FFAR4/GPR120 inhibition is at or proximal to the IKKβ and JNK kinases. TAK1 activation stimulates both the IKKβ/NF-κB and JNK/AP1 pathways, and the TLR and TNFα signaling pathways converge at this step. Stimulation of FFAR4/GPR120 has been shown to specifically inhibit TAK1 phosphorylation and activation, providing a common mechanism for the inhibition of both TLR and TNFα signaling.
Activation of FFAR4/GPR120 by DHA results in the association of the receptor with β-arrestin2. DHA stimulation results in the recruitment of β-arrestin2 to the plasma membrane where it co-localizes with FFAR4/GPR120. Following association between FFAR4/GPR120 and β-arrestin2 the complex is internalized and the complex is colocalized in the cytoplasmic compartment. TAB1 is the activating protein for TAK1 and following DHA-stimulated internalization of the FFAR4(GPR120)/β-arrestin2 complex, β-arrestin2 associates with TAB1 (TAK1 binding protein). The interaction of β-arrestin2 blocks the ability of TAB1 to associate with TAK1, thereby inhibiting TAK1 activation and downstream signaling to the IKKβ/NFκB and JNK/AP1 system. The anti-inflammatory effects mediated by FFAR4/GPR120 are entirely dependent on β-arrestin2. However, not all of the biological effects of DHA exerted via activation of FFAR4/GPR120 rely on β-arrestin2 association with the receptor.
FFAR4/GPR120 is expressed in mature adipocytes, but not preadipocytes. DHA stimulation of FFAR4/GPR120 in adipocyte precursor cells in culture results in increased GLUT4 translocation to the cell surface with a subsequent increase in glucose transport into the cells. The DHA-mediated effects on glucose uptake through FFAR4/GPR120 stimulation in adipocytes turns out to be independent of β-arrestin2. The effects of DHA on glucose uptake in adipocytes in culture are additive to those of a submaximally stimulating concentration of insulin.
Although it is possible to propose that the insulin sensitizing effects of omega-3 PUFA in adipocytes contributes to the overall insulin sensitizing actions of these fatty acids, muscle glucose uptake accounts for the great majority of insulin stimulated glucose disposal but FFAR4/GPR120 is not expressed in muscle.
In addition, experiments with muscle cells in culture demonstrate that DHA does not stimulate glucose uptake. However, since chronic, low grade tissue inflammation is an important cause of obesity-related insulin resistance, the anti-inflammatory effects of FFAR4/GPR120 stimulation are most likely coupled to insulin sensitizing actions in vivo.
Comparing effects of omega-3 PUFA in wild-type and FFAR4/GPR120 knock-out (KO) mice demonstrates the link between inflammation and insulin sensitivity. When fed a normal diet, lean FFAR4/GPR120 KO mice are glucose intolerant, hyperinsulinemic and they have decreased skeletal muscle and liver insulin sensitivity. These FFAR4/GPR120 KO mice also have a 2- to 5-fold higher level of expression of several proinflammatory genes. Feeding a high fat diet to both the FFAR4/GPR120 KO and the wild-type mice will result in obesity and insulin resistance. Of significance is the fact that when these mice are supplemented with omega-3 PUFA (such as DHA) the wild-type, but not the FFAR4/GPR120 KO mice, show a dramatic increase in insulin sensitivity.
In addition, omega-3 PUFA supplementation results in a decrease in adipose tissue markers of inflammation as well as anti-inflammatory effects in macrophages only in the wild-type mice. The in vivo anti-inflammatory actions of omega-3 PUFA are consistent with the insulin sensitizing effects of these lipids and are completely dependent on the presence of FFAR4/GPR120.
Lipid profile effects of omega-3 PUFA are also directly related to activation of FFAR4/GPR120. In wild-type and FFAR4/GPR120 KO mice fed a high fat diet there is an increase in total triglyceride, diglyceride, short-chain fatty acids, monounsaturated fatty acids (MUFA) and omega-6 fatty acids in the blood. All of these lipid changes are ameliorated with omega-3 PUFA treatment in wild-type but not FFAR4/GPR120 KO mice. Results such as these obtained in experimental animals are consistent with the view that the reversal of metabolic dysfunction-associated fatty liver disease (MAFLD) by omeag-3 PUFA treatment is mediated, in part, by activation of FFAR4/GPR120.
The results of numerous animal studies on the functions of omega-3 PUFA in inflammation, insulin sensitization, and lipid profiles mediated through activation of FFAR4/GPR120 indicates that this GPCR is a critically important control point in the integration of anti-inflammatory and insulin sensitizing responses, which may prove useful in the future development of new therapeutic approaches for the treatment of diabetes. However, there is some controversy regarding the direct anti-inflammatory effects of EPA and DHA being mediated through FFAR4/GPR120 activation since studies in mice in which the FFAR4 gene was knocked out still exhibited EPA- and DHA-mediated increases in insulin sensitivity and decreases in inflammatory responses.
Clinical Significance of Omega-3, and -6 PUFA
It is important to denote that when discussing omega-3 fatty acids, their dietary origin is quite important. Omega-3 fats from plants, such as those in flax seed oil, are enriched in ALA. As indicated above, ALA must first be converted to EPA (requiring three independent reactions) and then to DHA (requiring and additional four reactions). Omega-3 fats from cold water fishes and from Krill are enriched in EPA and DHA and thus, do not need to undergo the complex conversion steps required of ALA. In addition, the conversion of ALA to EPA and DHA is inefficient in general (discussed above) and is further compromised in individuals consuming a typical Western diet rich in animal fats as this type of diet impairs the functions of the both D5D and D6D enzymes required for ALA conversion to EPA and DHA.
When omega-3 and omega-6 fatty acids are consumed they are incorporated into cell membranes in all tissues of the body. Because of this fact, dietary changes in the composition of PUFA can have profound effects on a cell’s function because the membrane lipids serve as a source of precursors for the synthesis of important signaling molecules involved in cell growth and development as well as modulation of inflammation. Another important consequence of dietary alteration in fatty acid composition is the fact that omega-3 and omega-6 PUFA compete for incorporation into cell membranes.
The most important omega-6 PUFA is arachidonic acid. When cells are stimulated by a variety of external stimuli, arachidonic acid is released from cell membranes through the action of phospholipase A2 (PLA2). The released arachidonate then serves as the precursor for the synthesis of the biologically active eicosanoids, the prostaglandins (PG), thromboxanes (TX), and leukotrienes (LT).
The arachidonate-derived eicosanoids function in diverse biological phenomena such as platelet and leukocyte activation, signaling of pain, induction of bronchoconstriction, and regulation of gastric secretions. These activities are targets of numerous pharmacological agents such as the non-steroidal anti-inflammatory drugs (NSAID), COX-2 (PTGS-2) inhibitors, and leukotriene antagonists.
Dietary omega-3 PUFA compete with the inflammatory, pyretic (fever), and pain promoting properties imparted by omega-6 PUFA because they displace arachidonic acid from phospholipids present in cell membranes. In addition, omega-3 PUFA compete with the enzymes that convert arachidonic acid into the biological eicosanoids (PG, TX, and LT). The net effect of increasing dietary consumption of omega-3 PUFA, relative to omega-6 PUFA, is to decrease the potential for monocytes, neutrophils, and eosinophils (i.e. leukocytes) to synthesize potent mediators of inflammation and to reduce the ability of platelets to release TXA2, a potent stimulator of the coagulation process.
Although there is vastly more data on the beneficial effects of EPA and DHA, evidence has shown that docosapentaenoic acid (DPA) plays an important roll in reducing neuroinflammation and benefiting mental health outcomes. Studies carried out in animal models have shown that dietary supplementation with DPA exerts a positive effect on mental status, specifically with respect to depressive symptoms. Human studies have demonstrated a correlation between dietary DPA and reductions in major depressive disorder (MDD).
Receptors for EPA and DHA Derivatives
The various lipid mediators derived from EPA and DHA exert their effects by binding to cell surface receptors, the majority of which belong to the G-protein coupled receptor (GPCR) family. The various bioactive metabolites of DHA are known to bind to the cannabinoid receptors, the transient receptor potential (TRP) channels, N-formyl peptide receptor 2 (FPR2), GPR32, and adhesion G protein-coupled receptor F1 (also called GPR110). GPR32 has been shown to bind to RvD1 and to the lipoxin, LXA4. GPR32 is also activated by binding the D-series resolvins RvD3 and RvD5, and the aspirin-triggered epimer of RvD3, AT-RvD3. The FPR2 receptor also binds RvD1 and LXA4. The chemerin chemokine-like receptor 1 (encoded by the CMKLR1 gene; protein formerly identified as ChemR23) is activated by the E-series resolvins RvE1 and RvE2. The leukotriene B4 (LTB4) receptor, BLT1 (encoded by the LTB4R gene), is also activated by RvE1 and RvE2.
Resolvins, Protectins, Maresins
Probably the most important role of the omega-3 PUFA, EPA and DHA, is that they serve as the precursors for potent bioactive and anti-inflammatory lipids called resolvins (Rv), protectins (PD), and maresins (MaR). The synthesis and biological activities of the Rv, PD, and MaR is covered in greater detail in the Bioactive Lipid Mediators of Inflammation page. Aspirin triggers the synthesis of epimeric forms of the resolvins, and protectins which are designated by the prefix AT, such as in the case of the aspirin triggered PD1 epimer, AT-PD1.
More precisely, the Rv, PD, and MaR molecules do not inhibit the onset of inflammation but stimulate the resolution of the response resulting in the shutting off of an inflammatory process. Thus far at least nine resolvin molecules have been characterized along with two protectins and two maresin molecules. Collectively, these immune modulating EPA and DHA derivatives are referred to as specialized proresolving mediators, SPM.
The resolvins were initially identified by their ability to promote the resolution of the inflammatory cycle, hence the derivation of their names as resolvins. The resolvins are divided into two classes with the E-series resolvins (RvE1, RvE2, and RvE3) being synthesized from EPA and the D series resolvins (RvD1–RvD6) being derived from DHA. The resolvin family members share a 17-hydroxy residue that is introduced into DHA by the lipoxygenase, 15-LOX. The E series resolvins reduce inflammation, regulate polymorphonuclear neutrophil (PMN) infiltration by blocking transendothelial migration, reduce dendritic cell function (dendritic cells are potent antigen presenting cells which prime T cell-mediated inflammatory responses), regulate IL-12 production and lead to resolution of the inflammatory responses. Several of the resolvins are synthesized in epimeric form via the effects of aspirin.
The protectin, identified as (N)PD1 (where the N refers to neuroprotectin) is derived from DHA through the actions of the lipoxygenase, 15-LOX and then enzymatic hydrolysis. Aspirin can trigger the formation of an epimeric PD1 molecule identified as AT-PD1. The protectins (N)PD1 and AT-PD1 have been shown to exert potent inflammation resolving actions by inhibiting PMN (polymorphonuclear leukocytes) chemotaxis. An additional protectin identified as protectin DX is formed through the sequential actions of two lipoxygenase reactions and this protectin has been shown to inhibit platelet aggregation, inhibit the formation of reactive oxygen species (ROS), and to inhibit COX-2 activity in neutrophils.
The maresins, named maresin 1 (MaR1) and MaR2, are derived through the action of the lipoxygenase, 12-LOX on DHA. Maresin 1 is produced mainly by macrophages hence the derivation of the name maresin from macrophage mediator resolving inflammation. Maresin 1 exhibits potent anti-inflammatory, pro-resolving, analgesic and wound healing activities.
Major cellular targets for the actions of MaR1 are vascular smooth muscle (VSM) cells and vascular endothelial cells. In these cells MaR1 attenuates the adhesion of monocytes to the endothelium induced by tumor necrosis factor alpha (TNFα). Maresin 1 also inhibits the production of reactive oxygen species by both VSM and endothelial cells. The major mechanism through which MaR1 exerts these effects, in VSM and endothelial cells, is through down-regulation of the transcription factor, nuclear factor kappa-light-chain-enhancer of activated B cells (NF-κB). Maresin 2 has been shown to reduce neutrophil infiltration and to enhance macrophage-mediated phagocytosis of dead and dying cells, a process termed efferocytosis. Two related structures termed the maresin-like mediators (MaR-L1 and MaR-L2) are generated when the maresins produced by macrophages are released and acted upon by platelets.
Another important PUFA-derived inflammation resolving class of lipid mediator is the lipoxins (LX) which are derived from the omega-6 PUFA, arachidonic acid. The synthesis and biological activities of the lipoxins is covered in greater detail in the Bioactive Lipid Mediators of Inflammation page. Aspirin triggers the synthesis of epimeric forms of the lipoxins, as is the case for the effects of aspirin on the synthesis of epimeric resolvins and protectins.
Omega-3 PUFA and Brain Function
The omega-3 fatty acids DHA and EPA have also been shown to be important for normal brain development and function. Several studies have demonstrated that DHA is essential for proper development of the prenatal and postnatal central nervous system. The benefits of EPA appear to be in its effects on behavior and mood. In clinical studies with DHA and EPA there has been good data demonstrating benefit in treating attention deficit hyperactivity disorder (ADHD), autism, dyspraxia (motor skills disorder), dyslexia, and aggression. In patients with affective disorders consumption of DHA and EPA has confirmed benefits in major depressive disorder and bipolar disorder.
In addition, some studies have demonstrated promising results in treatment of schizophrenia with some minor benefits in patients with borderline personality disorder. Although originally purported to have positive effects in children with autism or autism spectrum disorder, recent clinical studies have shown that omega-3 PUFA supplementation does not have any positive effect on these disorders. Of significance to these effects of EPA and DHA on cognition, mood and behavior is the fact that administration of omega-3 fatty acid containing phospholipids (such as those present in Krill oils) are significantly better than omega-3 containing triglycerides such as those that predominate in fish oils.
Series 1 Eicosanoids from Omega Fats
Research over the past 10–15 years has demonstrated the physiological benefits (i.e. anti-inflammatory) of alternative pathways of polyunsaturated fatty acid metabolism. Much of this topic is covered in the Bioactive Lipid Mediators of Inflammation page. As described in the Eicosanoid Metabolism: Prostaglandins, Thromboxanes, Leukotrienes, and Lipoxins page, much of the DGLA derived from ingested linoleic acid or GLA is diverted into membrane phospholipids due to the inefficiency of the D5D enzyme catalyzing the conversion of DGLA to arachidonic acid.
Incorporation of DGLA into membrane phospholipids competes with the incorporation of arachidonate so that diets enriched in GLA result in an alteration in the ratio of membrane arachidonate to DGLA. Release of membrane DGLA occurs through the action of PLA2 just as for release of arachidonate. Once DGLA is released it will compete with arachidonate for COX and LOX enzymes.
The products of COX action on DGLA are series-1 prostaglandins (PGE1) and thromboxanes (TXA1). These eicosanoids are structurally similar to the series-2 eicosanoids except, of course, they have a single double bond. Although structurally similar, the series-1 eicosanoids have distinctly different biological actions. PGE1 and TXA1 are anti-inflammatory, they induce vasodilation, and they inhibit platelet aggregation.
When DGLA is a substrate for 15-LOX the product is 15-hydroxyeicosatrienoic acid (15-HETrE). 15-HETrE is a potent inhibitor of 5-LOX which is the enzyme responsible for the conversion of arachidonic acid to LTB4. LTB4 is a potent pro-inflammatory molecule through its action on neutrophils, thus, DGLA serves to inhibit inflammation via the linear eicosanoid pathway as well.
Due to the vasodilating action of PGE1 it is used pharmaceutically as aprostadil for the treatment of erectile dysfunction (ED). The ED applications of PGE1 are sold as MUSE® and Caverject®. MUSE is a urethral suppository and Caverject is an injectable version. Aprostadil is also used clinically to treat newborn infants with ductal-dependent congenital heart disease. The administration of aprostadil in these infants maintains a patent ductus arteriosus until surgery can be carried out to correct the underlying heart defect. Ductus arteriosus is a normal structure of the fetal heart that allows blood to bypass circulation to the lungs since the fetus does not use his/her lungs in utero. The ductus arteriosus shunts blood flow from the left pulmonary artery to the aorta. Shortly after birth the ductus closes due to the high levels of oxygen the newborn is exposed to at birth. However, in newborns with certain congenital heart defects, maintaining a patent ductus arteriosus is clinically significant.
Omega-3 PUFA and Regulation of Lipid Homeostasis
Omega-3 PUFA also are known to regulate hepatic lipid metabolism via regulation of the expression of key enzymes involved in lipid synthesis and catabolism. Omega-3 PUFA, primarily DHA, bind to and activate peroxisome proliferator-activated receptor-α (PPARα). Activation of PPARα by direct DHA binding results in activation of hepatic fatty acid oxidation. At the same time omega-3 PUFA inhibit hepatic fatty acid synthesis. This effect is mediated by suppression of SREBP-1c gene expression, enhanced degradation of SREBP1c mRNA and increased proteasomal degradation of SREBP-1 proteins. The decrease in SREBP-1c expression results in a reduction in the expression levels of hepatic fatty acid synthase (FAS) and acetylCoA carboxylase (ACC) two critical enzymes of fatty acid synthesis.
The net effect of fatty acid control of hepatic lipid metabolism is that the composition as well as the type and quantity of lipids available for VLDL synthesis and secretion are affected. Because the liver plays a central role in whole-body lipid metabolism this regulatory process in turn affects the lipid composition throughout the body and, therefore likely contributes to the onset and progression of several chronic diseases, including atherosclerosis, diabetes, and obesity.
Biological Activities of DHA Metabolites
Recent work has shown that the number of oxylipins derived from DHA is quite extensive and includes many more metabolites than just the resolvins, protectins, and maresins. Bioactive DHA-derived lipids include the fatty acid esters of hydroxy fatty acids, FAHFA (also see next section), the acylglycerols, the neuroprostanes (NP), the neuroketals, the ethanolamines, the electrophilic oxo-derivatives (EFOX), the epoxides, and the N-acyl amides.
DHA-Derived Fatty Acid Esters of Hydroxy Fatty Acids, FAHFA
Branched fatty acid esters of hydroxy fatty acids (FAHFA) are newly identified endogenous lipids regulated by fasting and high-fat feeding and associated with insulin sensitivity. FAHFA belong to the lipid class referred to as estolides which are intermolecular esters of fatty acids. The FAHFA were initially identified in mice in which expression of the GLUT4 gene was overexpressed specifically within adipose tissue. Their synthesis was shown to be regulated by both fatty acid content of the diet (e.g. high-fat diets) and by fasting. Strikingly, the level of synthesis of FAHFA isomers is highly correlated to insulin sensitivity with their synthesis being dramatically reduced in adipose tissue of humans exhibiting insulin resistance.
In humans the FAHFA isomers are found in numerous tissues as well as in the blood with highest concentrations being found in white and brown adipocytes. When FAHFA isomers were administered to mice it was observed that circulating levels of glucose declined while the levels of the gut and pancreatic hormone GLP-1 increased as did the level of insulin secretion.
Structurally, the initially characterized FAHFA isomers were found to be comprised of a C-16 or C-18 fatty acid either saturated or monounsaturated (e.g., palmitoleic, palmitic, oleic, or stearic acid) linked to a hydroxylated C-16 or C-18 lipid, again either saturated or monounsaturated. During the studies in which FAHFA were originally identified, palmitic-acid-9-hydroxy-stearic acid (9-PAHSA) was shown to be elevated the most in serum and adipose tissue of the GLUT4 overexpressing mice. This FAHFA is formed from palmitic acid esterification to 9-hydroxy stearic acid. When examining the presence of FAHFA in humans, 9-PAHSA was found to be the most predominant isomer. The levels of 9-PAHSA are reduced in the serum and adipose tissue in the insulin-resistant state of type 2 diabetes in humans.
Since their initial discovery (in 2014) several other FAHFA isomers have been identified such as those composed of DHA linked to carbon 9 or carbon 13 of the hydroxylated form of the essential fatty acid, linoleic acid and these compounds are identified as 9-DHAHLA and 13-DHAHLA (see Figure below). Another DHA containing FAHFA contains DHA esterified to 14-hydroxy DHA and is identified as 14-DHAHDHA. The level of synthesis of DHA containing FAHFA in white adipose tissue (WAT) is similar to the level of protectins and resolvins derived from DHA in WAT. The activity of 13-DHAHLA has been shown to be both anti-inflammatory and proresolving.
Although the enzymes involved in the synthesis of the various FAHFA are not yet fully characterized, two enzymes that are FAHFA-specific hydrolases have recently been identified. Although not previously characterized as hydrolytic enzymes these two proteins have now been shown to represent a novel class of threonine hydrolases that are involved in the metabolism of bioactive lipids. These two enzymes are both multipass transmembrane proteins and they were originally identified as androgen induced 1 (encoded by the AIG1 gene) and its sequence-related homologous protein called androgen dependent tissue factor pathway inhibitor (TFPI) regulating protein (encoded by the ADTRP gene).
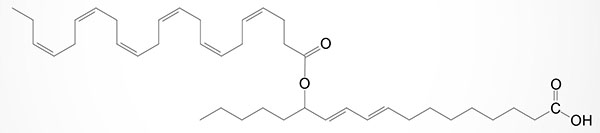
DHA-Derived Neuroprostanes, NeuroP
The DHA-derived neuroprostanes (NeuroP) are prostaglandin-like compounds formed as a result of free radical nonenzymatic peroxidation and cyclization of DHA. The initial peroxidation of DHA can occur at carbon 6, 9, 12, 15, or 18, relative to the carboxylic acid group, resulting is the potential for a large number of different NeuroP isoforms (A4-, D4-, E4-, F4-, and J4-neuroprostanes). Although a large number of NeuroP are possible, the forms identified in vivo are the F-type (two hydroxyl groups on ring) prostane ring forms (F4-NeuroP) and these NeuroP belong to the 4-, or 20-series regioisomers (positional isomers), which are dependent on the position of the hydroxyl group not associated with the prostane ring (see Figure below). The A4– and J4-NeuroP isoforms contain a single carbonyl oxygen (=O) attached to the prostane ring, whereas the D4– and E4-NeuroP isoforms contain a carbonyl oxygen and a hydroxyl group attached to the prostane ring. The NeuroP (particularly the F4-NeuroP isoforms) have been shown to exert significant cardioprotective activity including a reduction of abnormal events such as arrhythmias and systolic cardiac failure. The A4– and J4-NeuroP isoforms have been shown to exhibit anti-inflammatory activities with 14-A4-NeuroP having been shown to inhibit NF-κB-mediated signaling in macrophages, thereby suppressing inflammatory responses in these cells.
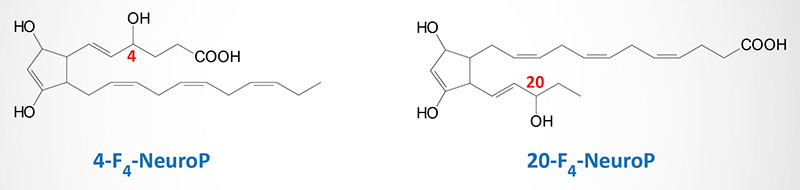
DHA-Derived Ethanolamines
DHA is highly enriched in the brain and several lines of evidence indicate that normal brain development is dependent upon this omega-3 PUFA. Within the brain DHA has been shown to be metabolized to an endocannabinoid-like ethanolamine derivative called synaptamide (N-docosahexaenoyl ethanolamine, DHEA).
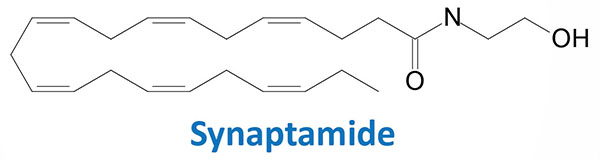
Synaptamide is most likely synthesized from DHA-containing phosphatidylethanolamine (DHA-PE) with the eventual release of synaptamide by a specific phospholipase D (PLD). Synaptamide has been shown to promote neurite outgrowth, to promote the differentiation of neural stem cells, and to enhance glutamatergic synaptic activity. Synaptamide also exhibits the ability to reduce neuroinflammation and to reduce inflammatory responses of macrophages, and to exert beneficial metabolic effects in adipose tissue. The effects of synaptamide in adipose tissue is likely the result of the activation of PPARα.
DHA-Derived Electrophilic Oxo Derivatives, EFOX
Electrophilic oxo derivatives (EFOX) of DHA (as well as many other lipids) can be generated via both enzymatic and non-enzymatic pathways. Numerous EFOX compounds have been shown to act as ligands for PPARγ. The compounds 17-oxo-DHA and 13-oxo-DHA are generated in macrophages by a mechanism catalyzed by COX-2. Both of these DHA EFOX derivatives have been shown to act as agonists of PPARγ and to inhibit pro-inflammatory cytokine and nitric oxide (NO) production by macrophages. In human neutrophils the lipoxygenase, 5-LOX, generates 7-oxo-DHA. Another DHA EFOX derivative, 4-oxo-DHA, has been shown to exhibit anticancer activity via inhibition of cancer cell proliferation.
DHA-Derived Epoxides
The xenobiotic metabolizing cytochrome P450 epoxygenase enzyme, CYP2J2, can convert DHA to the epoxide derivative, epoxydocosapentaenoic acid (EpDPE; sometimes designated EDP or EpDPA). There is a family of EpDPE compounds that are formed by differential incorporation of the epoxide. Within the EpDPE family of compounds the 19,20-EpDPE (19,20-epoxy-DPA) compound has been shown to exert the highest level of bioactivity. This EDP compound acts as a mediator the anti-hypertensive effects associated with ingestion of DHA and the compound is also a potent antiarrhythmic agent. 19,20-EDP has also been shown to inhibit vascular endothelial growth factor (VEGF)- and fibroblast growth factor 2 (FGF2)-induced angiogenesis, tumor growth, and metastasis. Within the liver an elevated level of 19,20-EpDPE has been associated with a reduction of lipid peroxidation and hepatic inflammation.
DHA-Derived N-acyl Amides
The secreted enzyme identified as peptidase M20 domain containing 1 (PM20D1) catalyzes the N-acylation of a variety of amino acids forming N-acyl amides. Several lines of evidence have demonstrated that N-acyl amides improve glucose homeostasis and overall energy metabolism, and several act as endogenous uncouplers of mitochondrial oxidative phosphorylation. Several DHA containing N-acyl amides have been identified (N-DHA-Ser, -Gly, -Val, -Asp, -Tyr, -Trp, and -Pro) and shown to be modulators of the activity of the transient receptor potential vanilloid (TRPV) channels.